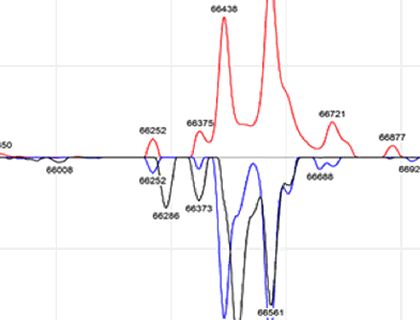
Albumin in Cell Culture Media – An examination of quality and function
Part 2 of the Cell Culture Media Optimization Series
A Guest Blog by Randall Alfano, Ph.D., Vice President Product Development, InVitria
Albumin plays many roles in the human body and can provide several benefits when added to cell culture media. In large part, the albumin found in most cell culture is sourced from human serum. Human serum derived albumin presents many potential issues for cell culture scientists, including inconsistency and the possible introduction of adventitious agents. However, the successful substitution of human serum-derived albumin with recombinant albumin can be involved due to the inherent complexity of the albumin protein and its associated broad functionality both in vivo and in vitro. Thus, an in-depth examination of the biology of albumin is required prior to addressing function in cell culture applications.
Background
Due to their longer sequence and sophisticated protein structure, human serum transferrin and albumin represent major challenges for recombinant protein expression and successful implementation in downstream cell culture applications. As previously discussed, the similar biochemical characteristics of the recombinant version of transferrin translated into identical function when compared to native human serum-derived transferrin (Zhang D 2010; Steere AN 2012). This allows for a relatively straightforward replacement of human serum-derived transferrin by the animal free recombinant Optiferrin. On the other hand, the complex nature of albumin creates more issues that need to be considered when choosing an albumin to add to cell culture processes.
Human Serum Albumin Structure and Function
The ~66 kD serum albumin is one of the most abundant proteins found in the plasma of all vertebrates (Lee P 2015). The single-chain nonglycosylated polypeptide that forms the primary structure of albumin folds into mostly α-helices with an overall structure that resembles a heart (Yang F 2014). Cumulatively, serum albumin consists of 9 double loops that span 3 homologous domains dubbed Domain I-III. Each of these 3 domains consists of 2 long loops (subdomain A) and 1 short loop (subdomain B).
Human serum albumin has evolved to have primary roles in many different important biological processes. Due to the high concentration of albumin in plasma (35-50 mg/mL), this protein is responsible for the majority of colloidal osmotic pressure of the circulatory system (Taverna M 2013). Further, albumin can bind a multitude of molecules including ions, fatty acids, steroids, and drugs, and has been used extensively for direct targeting of therapeutics and enhancing pharmacokinetics as a result (Sleep D 2013; Lee P 2015). Interestingly, each domain within serum albumin demonstrates preferential binding to different substrates. Albumin in itself is a potent antioxidant, waste carrier, and reactive oxygen/nitrogen species scavenger and has been used as a therapeutic agent for shock, acute management of burns, and other hypovolemic-associated conditions (Taverna M 2013).
Human Serum Albumin in Cell Culture Media
Given the multifaceted functional aspects of albumin, it’s no surprise that the addition of this protein greatly enhances the growth and viability of mammalian cells when cultured ex vivo for extended periods of time. The aforementioned abundance of serum albumin in plasma has enabled the extraction and purification of this protein for use in mammalian cell culture. Indeed, human serum albumin represents a multimillion dollar industry for cell culture alone and a plethora of commercially available xeno-free media incorporate albumin sourced from pooled human serum.
However, like serum-derived transferrin, sourcing albumin from human serum for downstream cell propagation brings inherent risk of variability amongst lots/preparations and vendors that could translate to unpredictable downstream final product quality. Previous studies have uncovered variability in the addition of stabilizers by different human serum albumin suppliers (Leonard P 2013). Addition of stabilizers, such as octanoic acid, has been shown to have profound negative effects in specific cell culture systems. Further, additional studies found variable oxidation states in human serum albumin lots intended for clinical use when compared to fresh isolations of healthy volunteers (Bar-Or D 2005). Other significant posttranslational differences in commercial human serum-derived albumin preparations were also found to variable degrees (Bar-Or D 2005).
Raw material homogeneity aside, inclusion of blood sourced components into cell culture media carries an additional risk of potential contamination of the final product by adventitious agents (O 1999; Broedel S 2003; Hulse W 2013; Usta S 2014). Even if viral testing is conducted on albumin lots prior to utilization in cell culture systems, complete risk mitigation cannot be achieved due to new or emerging pathogens that may not currently be considered for testing (Belay E 2005).
The importance of the risks associated with utilization of serum-derived albumin warrants the consideration of a recombinant alternative for inclusion in cell culture media. Successful production of recombinant human albumin and subsequent commercialization has been achieved. We have successfully expressed and purified two different recombinant albumins in a highly scalable, animal-free expression host. The first albumin, Cellastim, is a highly pure, lipid rich human albumin that is composed of approximately 0.8% lipids by mass. The second, known as Optibumin, has comparable purity to that of Cellastim, but is nearly lipid-free. Before examining the functionality of serum-derived albumin and how it compares to recombinant versions, it is critical to understand the analytical differences between commercially available serum-derived and recombinant albumins to gain a working knowledge of raw material quality. The functional analysis and comparison of serum-derived albumin to other commercial albumins from different sources will be the subject of future discussion.
Gaining an Analytical Perspective of Commercially Available Albumins
In order to assess the analytical characteristics of currently marketed albumin products, we compared two different sources of serum-derived albumin, two recombinant yeast-derived albumins, the lipid rich Cellastim, and the lipid poor Optibumin via mass spectrometry and reverse phase HPLC (identity) as well as SEC-HPLC and Native-PAGE (purity).
Mass spectrometry determined that Cellastim exhibits two prominent peaks that correspond to the mercapto-albumin species (66,438 Da) and the mono-cysteinylated albumin species (66,559 Da). This LC-MS profile was noted to be very similar to that of both native albumins (Figure 1A) while the yeast-derived albumins present only a single 66439 Da peak (Figure 1B). Interestingly, the lipid poor Optibumin exhibited a very similar LC-MS profile to that of the yeast-derived recombinant albumin in that one prominent 66439 Da peak was noted and identified as the mercapto-albumin species (Figure 1C). Collectively, Cellastim appears to be very similar to serum-derived albumin while the Optibumin and the yeast derived albumins are significantly different by LC-MS.
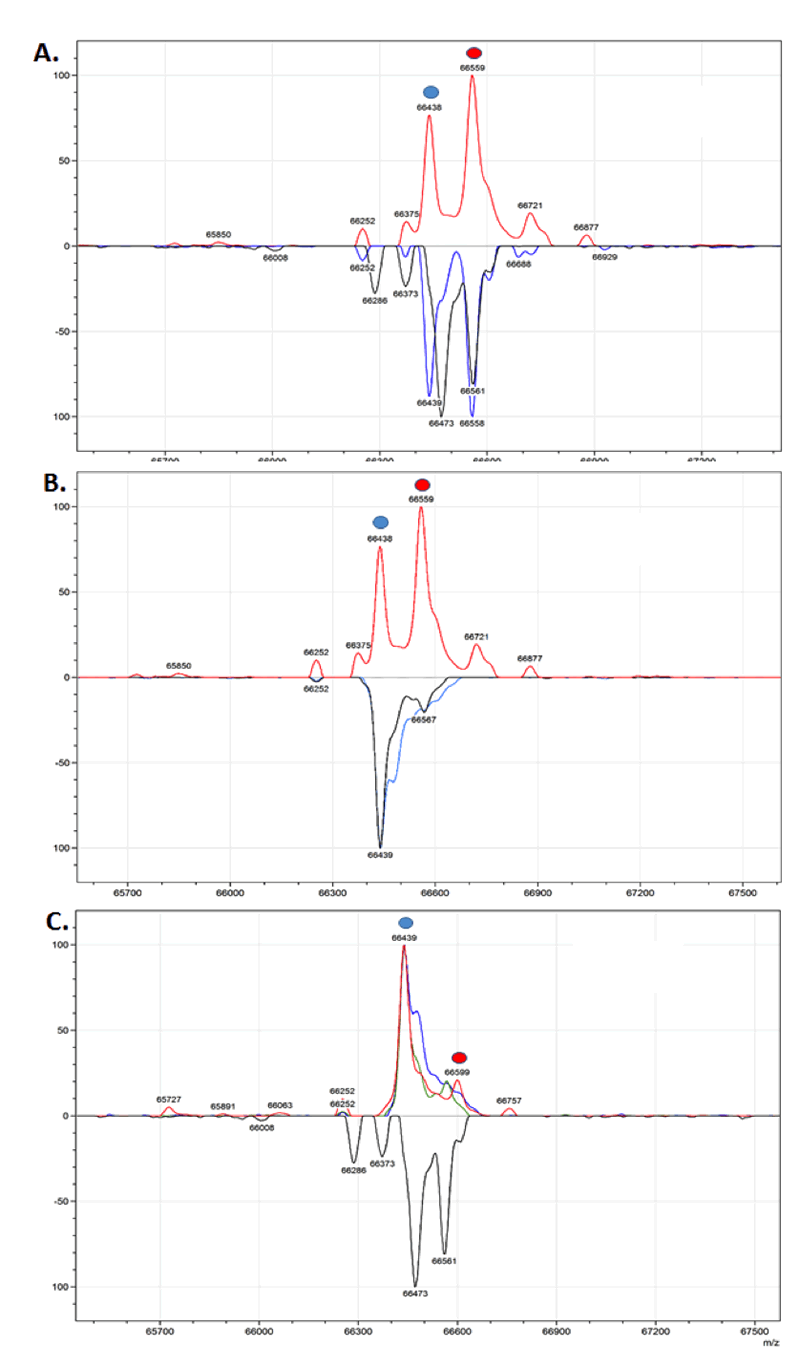
Figure 1. Liquid Chromatography Mass Spectrometry Profile of Albumins.
(A) Cellastim (red trace) demonstrated two prominent peaks that were identified as the 66,438 Da mercapto-albumin (blue dot) and the 66,559 Da mono-cysteinylated albumin species (red dot). This profile was noted to be very similar to the two human serum-derived albumins (blue and black traces). (B) Cellastim (red trace) was noted to be very different from the two yeast-derived recombinant albumins (black and blue traces) where only a single major peak was identified as mercapto- albumin species. (C) Optibumin (red trace) demonstrated a single prominent peak that corresponded to the 66,439 Da mercapto-albumin species (blue dot) and only a minor population identified as the 66,559 Da mono-cysteinylated albumin species (red dot). This was noted to be very similar to the yeast-derived albumins (green and blue traces) but significantly different from serum-derived albumin (black trace).
Reverse phase HPLC further supported the identity trends observed in the LC-MS data. Cellastim was found to have a very similar profile to those of the serum-derived albumins (Figure 2A), but profoundly different than those of the yeast-derived albumins (Figure 2B). As expected, Optibumin had a very similar profile to those of the yeast-derived albumins, but significantly different from those of serum-sourced (Figure 2C).
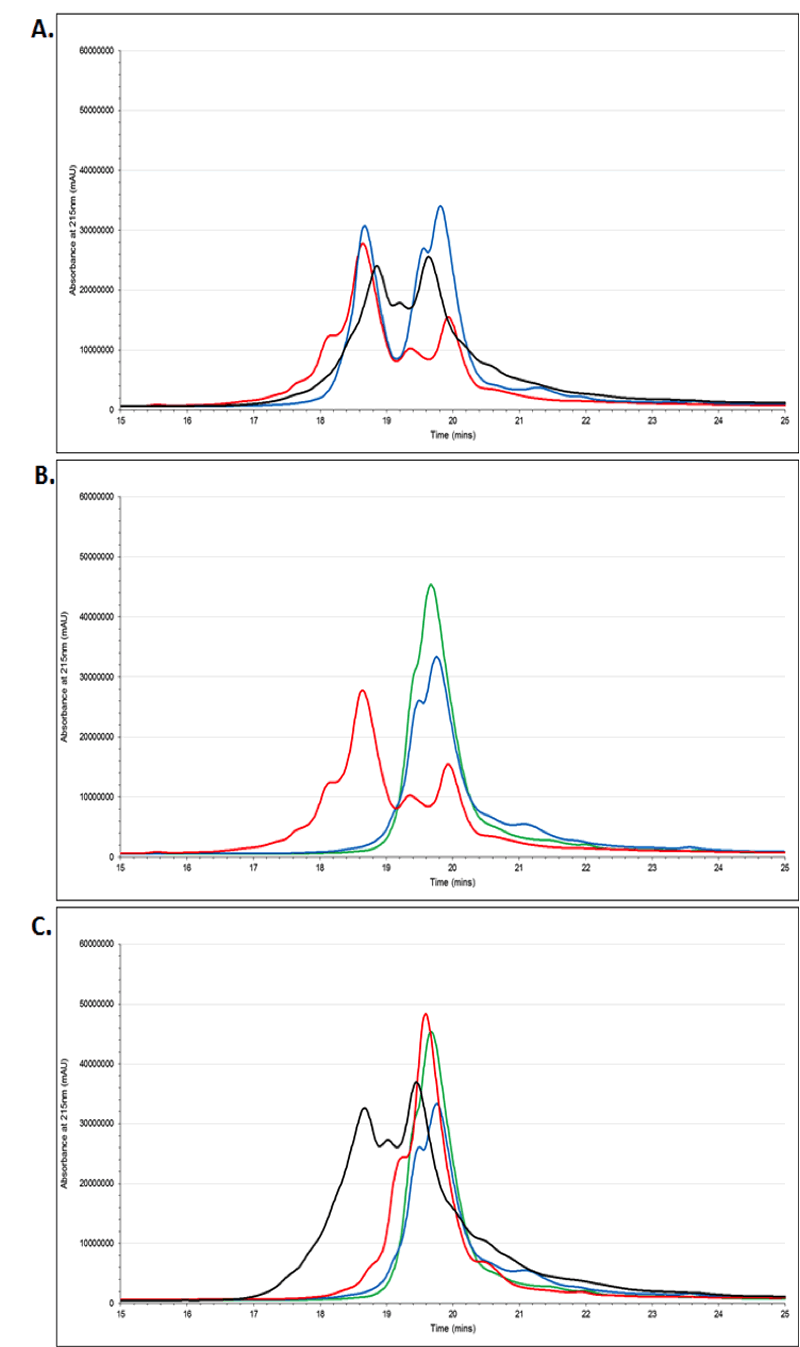
Figure 2. Reverse Phase HPLC Analysis of Albumins.
(A) Cellastim (red trace) exhibited a very similar chromatographic profile to those of serum-derived albumins (blue and black trace) from different vendors. (B) Cellastim (red trace) was found to be significantly different from yeast-expressed recombinant albumins (blue and green traces) while (C) Optibumin (red trace) demonstrated an equivalent chormotographic profile to the yeast derived albumins (blue and green traces) but very different from serum-derived albumin (black trace).
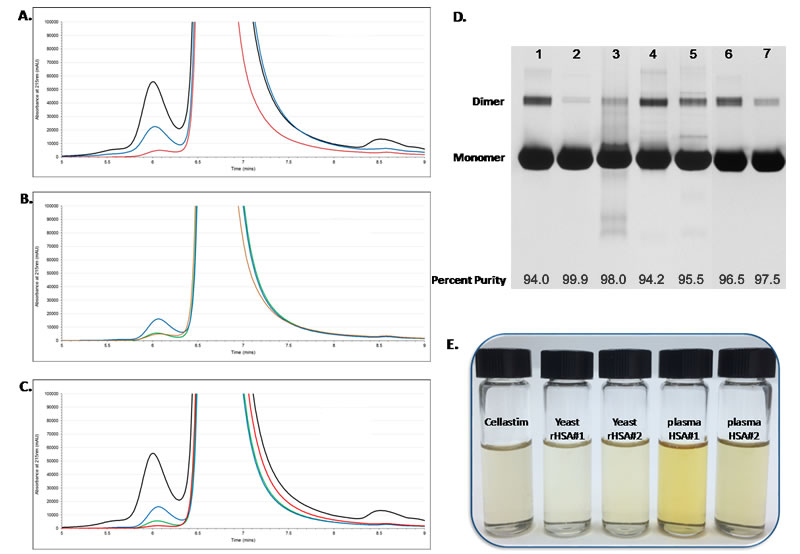
Figure 3. Monomer Purity and Color of Recombinant Albumins Cellastim and Optibumin.
Cellastim and Optibumin were analyzed by SEC-HPLC and Native-PAGE analysis in order to determine monomer purity. (A) Cellastim (red trace) exhibited fewer high molecular weight aggregates than either of the serum-derived albumins (blue and black traces). (B) Cellastim (red trace) had comparable high molecular weight aggregates to one of the yeast-derived albumins (green trace) and fewer than the other (blue trace). (C) Optibumin (red trace) exhibited fewer high molecular weight aggregates than either of the yeast-derived albumins (blue and green traces) and the serum-derived albumin (black trace). (D) Native-PAGE analysis of albumins further reinforced the purity of Cellastim and Optibumin observed in the SEC-HPLC analysis. Quantitative Native-PAGE was carried out according to USP monograph of rHSA. Gel order is as follows: Lane 1: USP standard, Lane 2: Optibumin, Lane 3: Cellastim, Lane 4: Serum-derived albumin vendor 1, Lane 5: Serum-derived albumin vendor 2, Lane 6: Yeast-derived recombinant albumin 2, Lane 7: Yeast-derived recombinant albumin 1. Monomer purity is indicated at the bottom of the gel. (E) Cellastim was dissolved into a 20% solution using cell culture-grade water and compared to 20% solutions of yeast- or serum-derived albumins. Serum-derived albumins were adjusted to 20% using cell culture-grade water.
Purity was determined via SEC-HPLC as well as Native-PAGE. Cellastim exhibited a very similar profile to the serum derived albumins, albeit with fewer high molecular weight aggregates (Figure 3A). and was comparable to that of the yeast derived albumins (Figure 3B). Similarly, Optibumin was found to have fewer high molecular aggregates than both native albumins as well as both of the yeast-derived albumins (Figure 3C). Indeed, Native-PAGE indicated a very high purity of Cellastim and Optibumin at 99 and 100% monomer purity, respectively, compared to the USP standard as well as both serum-derived albumins at 96 and 98% (Figure 3D). Further, the comparable color of Cellastim (Figure 3E) and Optibumin (not shown) to yeast-derived recombinant albumins indicates a lack of organic contaminates.
Conclusions and Final Remarks
The performance of any given serum free cell culture media ultimately depends on not only the media composition but also the quality of reagents incorporated. Determining which type of albumin is appropriate for a desired application requires a working knowledge of the analytical differences between human serum-derived albumin and recombinant versions. Here, we demonstrated that the lipid rich Cellastim exhibits a comparable profile to serum-derived albumins by LC-MS and RP-HPLC. Cellastim also showed fewer high molecular weight aggregates by SEC-HPLC and Native-PAGE, and fewer contaminating undefined organic macromolecules by color assessment. Recombinant albumins derived from yeast, however, exhibited significantly altered profiles by LC-MS and RP-HPLC, but had similar monomer purity when compared to Cellastim. On the other hand, Optibumin exhibited a comparable profile to yeast-derived albumins by LC-MS and RP-HPLC, and showed fewer high molecular weight aggregates as determined by SEC-HPLC and Native-PAGE.
From these analytical results, recombinant albumins demonstrated significantly increased purity compared to serum-derived products. This superiority in raw material quality, combined with the aforementioned risks of using human serum-derived components for downstream cell culture applications, suggests the inclusion of these recombinant proteins would be justified, subject to confirmation of functional activity. Analytical properties of any raw material are not necessarily predictive of function. Further, the ability of albumin to transport other biological molecules, such as fatty acids, convolutes any possible predictive capacity gained by the favorable interpretation of the analytical data. Subsequent studies will focus on the in vitro functional differences demonstrated by Cellastim and Optibumin, compared to serum-derived albumins and the consequences for cell growth and productivity in vitro.
Don’t miss Part I of the Cell Culture Media Optimization Series – “Generating Recombinant Versions of Human Serum-Derived Proteins – Transferrin and Albumin”
References
- Bar-Or D, B.-O. R., Rael L, Gardner D, Slone D, Craun M (2005). “Heterogeneity and oxidation status of commercial human albumin preparations in clinical use ” Crit Care Med 33(7): 1638-1641.
- Belay E, S. L. (2005). “Creutzfeldt-Jakob disease surveillance and diagnosis.” Dis(41): 834-836.
- Broedel S, P. S. (2003). “The Case for Serum-Free Media.” BioProcess Int(56).
- Hulse W, G. J., Forbes R (2013). “Evaluating the inter and intra batch variability of protein aggregation behaviour using Taylor dispersion analysis and dyanmic light scattering.” Int J Pharm 453(2): 351-357.
- Lee P, W. X. (2015). “Review: Modifications of Human Serum Albumin and Their Binding Effect.” Curr Pharm Des 21(14): 1862-1865.
- Leonard P, C. M., Benson L, Walker D, Fredrickson J, Morbeck D (2013). “Variability in protein quality used for embryo culture: embryotoxicity of the stabilizer octanoic acid.” Fertil Steril 100(2): 544-549.
- O, M. (1999). “Safety issues of animal products used in serum-free media.” Dev Biol Stand(99): 167-180.
- Sleep D, C. J., Evans LR (2013). “Albumin as a versatile platform for drug half-life extension.” Biochim Biophys Acta 1830(12): 5526-5534.
- Steere AN, B. C., Zhang D, Pettit SC, Kaltashov IA, Huang N, Mason AB (2012). “Biochemical and structural characterization of recombinant human serum transferrin from rice (Oryza sativa L.).” J Inorg Biochem(116): 37-44.
- Taverna M, M. A., Mira JP, Guidet B (2013). “Specific antioxidant properties of human serum albumin.” Ann Intensive Care 3(1).
- Usta S, S. C., Xu J, Frey T, Nash R (2014). “Chemically defined serum-free and xeno-free media for multiple cell lineages.” Ann Transl Med 2(10).
- Yang F, Z. Y., Liang H (2014). “Interactive Association of Drugs Binding to Human Serum Albumin ” Int J Mol Sci 15(3): 3580-3595.
- Zhang D, N. S., Bryan P, Pettit S, Nguyen D, Santos MA, Huang N (2010). “Expression, purification, and characterization of recombinant human transferrin from rice (Oryza sativa L.).” Protein Expr Purif 74(1): 69-79.