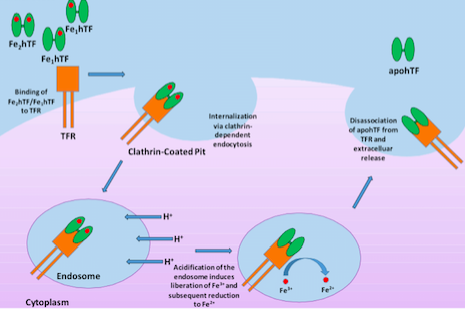
Generating Recombinant Versions of Human Serum-Derived Proteins – Transferrin and Albumin
Comparative Analysis of Biochemical Structure and Function
A Guest Blog by Randall Alfano, Ph.D., Vice President Product Development, InVitria
The deciphering of man’s genetic code followed by the advent of recombinant DNA technology has provided the scientific community with the ability to genetically manipulate and express a plethora of proteins for both research and therapeutic purposes. However, successful expression of any given protein does not always translate to acceptable or similar function when compared to the native version and thus can lead to unintended consequences in downstream applications.
In this series of blogs, we’ll examine this aforementioned issue in the context of cell culture media using human serum transferrin and albumin as specific examples. The successful expression of recombinant transferrin and albumin, their biochemical and analytical characteristics, and functionality in serum free media will be examined in detail in addition to further modifications that can enhance utility of these recombinant proteins.
Human Serum Transferrin and Albumin in Serum Free Media
Traditionally, cell culture media has consisted of a basal media mixed with 10-20% fetal bovine serum (FBS)1. Initial attempts to replace FBS with a completely defined formulation were met with the realization that certain proteins would have to be purified out of serum and added back to the formulation at optimal concentrations2. Currently, most serum free media suitable for clinical applications contain albumin and transferrin that are purified from human serum while other critical serum protein components, such as insulin and required cytokines, are frequently recombinant versions. This notable discrepancy is due to the fact that insulin and cytokines can be expressed efficiently and economically either by E. coli or other simpler eukaryotic expression systems such as yeast due the relatively short amino acid sequence and straightforward tertiary structure. Albumin and transferrin, however, are much more complex in size and structure. Further, these two proteins are required at relatively high concentrations in serum free media formulations. Thus, cumulatively, these proteins pose a greater challenge for expressing recombinantly at high levels and represent a major hurdle in the industry’s efforts to completely remove all human sourced components from media formulations.
Transferrin
Human serum transferrin, a bilobal ~75 kD glycoprotein that has the ability to reversibly bind Fe3+ with nanomolar affinity, represents one of the major vehicles for iron delivery to cells both in vivo and in vitro3. Produced in the liver, transferrin is found in the blood plasma as a heterogeneous population at approximately 2.5 mg/mL consisting of diferric (holo or iron-saturated), monoferric N-lobe, monoferric C-lobe (partially iron saturated) or apo transferrin4. At pH 7.4, the pH of human serum, holo transferrin has the highest affinity for the cell surface transferrin receptor (TFR) followed by the two monoferric forms4. One of these three iron-bound forms of transferrin will bind the TFR and be internalized via clathrin-dependent endocytosis5. Subsequent acidification of the endosome via ATP-dependent H+ pumps will trigger the receptor-stimulated release of iron from transferrin where the reduction of Fe3+ to Fe2+ ensues4. The now iron free transferrin/TFR complex is subsequently redirected back to the cell surface where the weak association for the TFR at serum pH will trigger the dissociation of apo transferrin so that apo transferrin can bind additional Fe3+ 6 (Figure 1). This process occurs quite rapidly so that each transferrin molecule delivers several payloads of iron per hour4. Although the fully saturated diferric transferrin has the highest affinity for the TFR (Kd ~4 nM), the fact that monoferric forms also are capable of high affinity bonds with the TFR (Kd ~36 and 32 nM for the FeNhTF and FeChTF, respectively) would indicate that partially iron saturated transferrin would be functional in cell culture4.
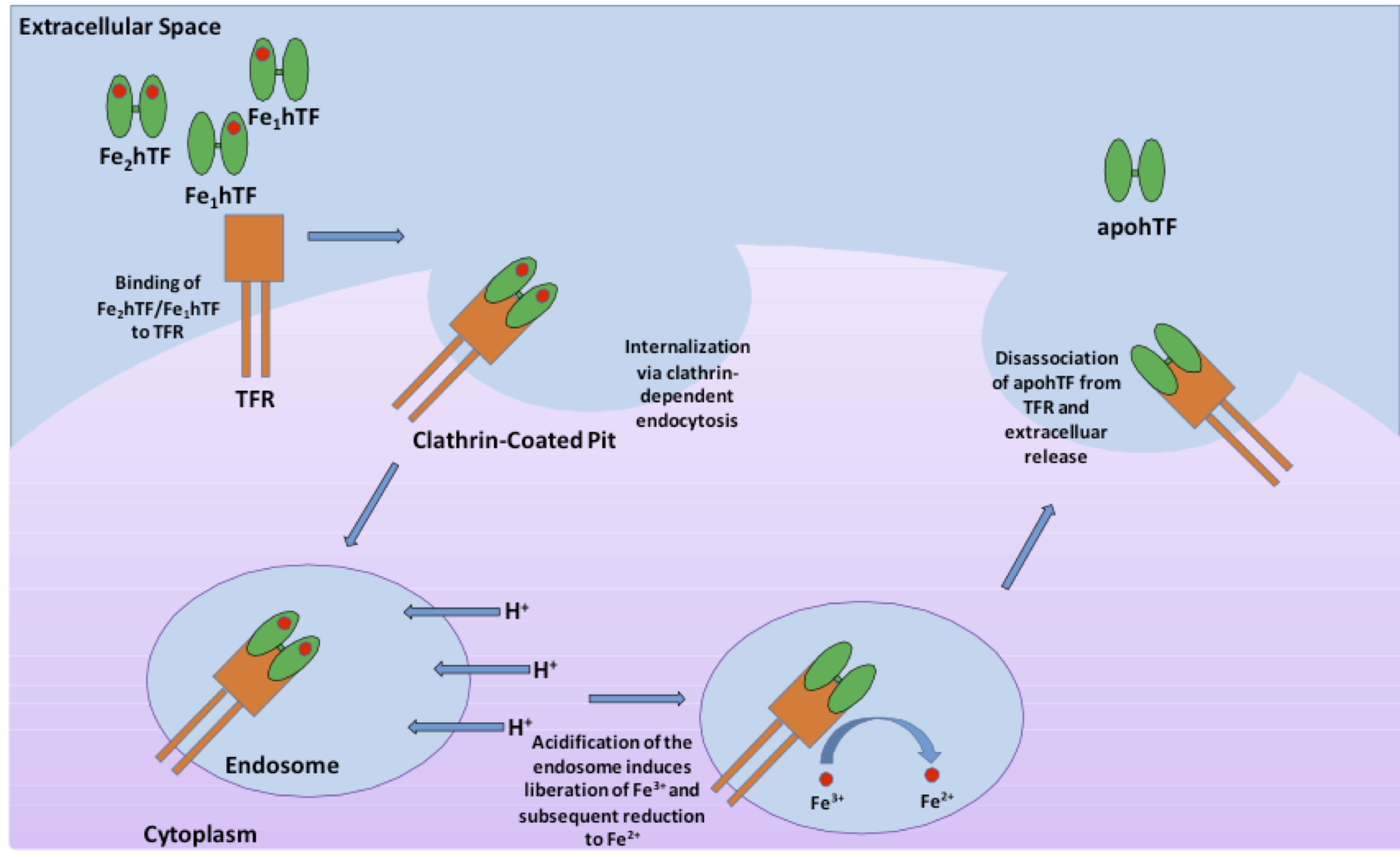
Given the central role of iron uptake in cell health, the inclusion of transferrin is absolutely critical in serum free cell culture media to ensure adequate cell proliferation and function ex vivo for most cell types. The natural abundance of transferrin in human serum combined with relatively straightforward purification has enabled the isolation and utilization of serum-derived transferrin for in vitro cell culture applications for years. However, given the inherent reliability issues and potential safety concerns of using serum-derived proteins, there have been extensive efforts to generate recombinant versions of human serum transferrin. Initial attempts using prokaryotic and simple eukaryotic expression systems were met with only limited success7,8. Low expression and/or expression of nonfunctional protein prompted the investigation of using more complex eukaryotic expression systems such as Baby Hamster Kidney (BHK)9,10.
We have successfully expressed recombinant human transferrin in an animal component free (ACF) host11. Interrogation of the biochemical and functional aspects of this recombinant transferrin indicated acceptable characteristics of the recombinant protein11. We found that this recombinant transferrin, called Optiferrin, possessed the correct sequence and size and was functionally similar. Recombinant transferrin was able to compete with serum-derived transferrin for TFR binding sites on both CCL-2 and Caco-2 cells11,12. As a result, cell proliferation was found to be identical in a hybridoma cell line across a range of relevant concentrations (Figure 2).
Animal Component Free, Recombinant Human Serum Transferrin Exhibits Broad Activity in Cell Culture
Given the identical structure and function of Optiferrin to serum-derived transferrin, it would be expected that Optiferrin would have utility across multiple cell systems and different serum free media formulations. Indeed, to date we have confirmed the activity of Optiferrin in human mesenchymal stem cells from multiple tissues, neural stem cells, induced pluripotent stem cells, primary T cells, fibroblasts from multiple tissues, keratinocytes, VERO cells, hematopoietic stem cells, and hybridoma cells. Further, the adoption of Optiferrin in industry has been steadily increasing since this recombinant protein debuted in 2012.
Substitution of serum-derived transferrin for Optiferrin in a serum free media is straightforward and can be expected to be nearly 1:1 if the concentration of native transferrin is known. If the concentration of native transferrin is not known, typical Optiferrin concentration ranges that have demonstrated adequate cell proliferation in the aforementioned cell types range from 10-400 mg/L and optimal concentration will have to be empirically determined.
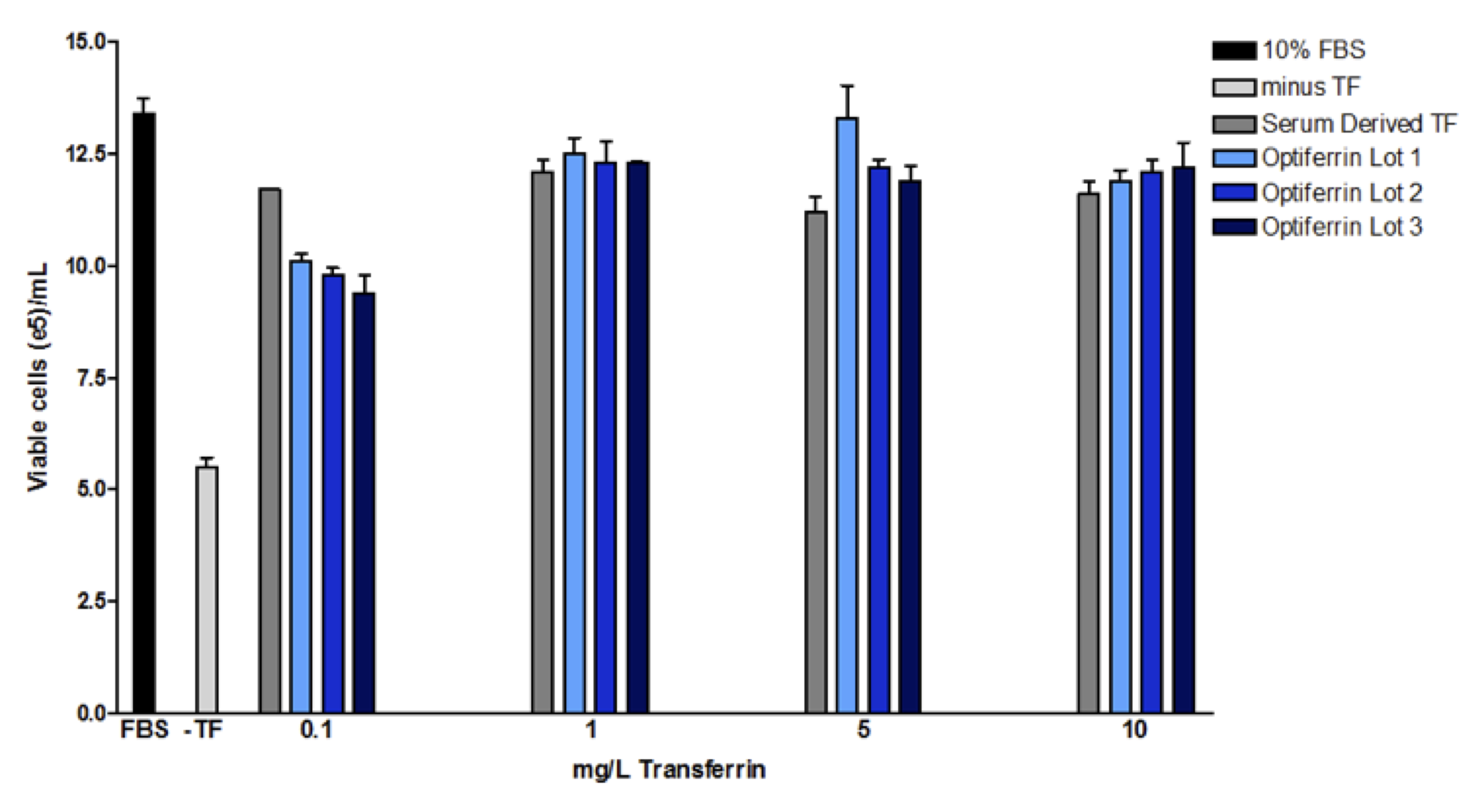
Final Remarks
The elucidation of recombinant DNA technology has given the scientific community great insights into the intricacies of protein structure and function. Yet, even after a genetic sequence is obtained and expressed within a recombinant host, there is still the challenge of demonstrating equivalence to the native protein. In the case of transferrin, the recombinant transferrin demonstrated structural and functional similarity to the serum-derived protein. However, serum albumin, the second protein mentioned, is a bit more complex in terms of both the biochemical characteristics and implementation of recombinant versions in cell culture media. This will be the topic of subsequent blogs.
References
- K, B. (2013). Platelet lysate as replacement for fetal bovine serum in mesenchymal stromal cell cultures. Transfus Med Hemother, 326-35.
- MCKeehan WL, B. D. (1990). Frontiers in mammalian cell culture. 26(1).
- Steere A, B. C. (2012). Biochemical and Structural Characterization of Recombinant Human Serum Transferrin from Rice (Oryza Sativa L.). J Inorg Biochem, 37-44.
- Luck AN, M. A. (2012). Transferrin-Mediated Cellular Iron Delivery. Current Topics in Membranes, 69, 3-35. doi:10.1016/B978-0-12-394390-3.00001-X
- Morgan EH, A. T. (1969). Autoradiographic localization of 125-I-labelled transferrin rabbit reticulocytes. Nature, 1371-2
- Leverence R, M. A. (2010). Noncanonical interactions between serum transferrin and transferrin receptor evaluated with electrospray ionization mass spectrometry. Proc Natl Acad Sci USA, 8123-8.
- Ikeda RA, B. B. (1992). Production of human serum transferrin in Escherichia coli. Gene, 265-9.
- Steinlein LM, I. R. (1993). Production of N-terminal and C-terminal human serum transferrin in Escherichia coli. Enzyme Microb Technol, 193-9
- Funk WD, M. R. (1990). Expression of the amino-terminal half-molecule of human serum transferrin in cultured cells and characterization of the recombinant protein . Biochemistry, 1654-60.
- Mason AB, H. P. (2004). Expression, purificaiton, and characterization of authentic monoferric and apo-human serum transferrins. Protein Expr Purif, 318-26.
- Steere AN, B. C. (2012). Biochemical and Structural Characterization of Recombinant Human Serum Transferrin from Rice (Oryza sativa L.). J Inorg Biochem, 37-44.
- Zhang D, L. H. (2012). Characterization of transferrin receptor-mediated endocytosis and cellular iron delivery of recombinant human serum transferrin from rice (Oryza sativa L.). BMC Biotechnology, 12, 92. doi:10.1186/1472-6750-12-9