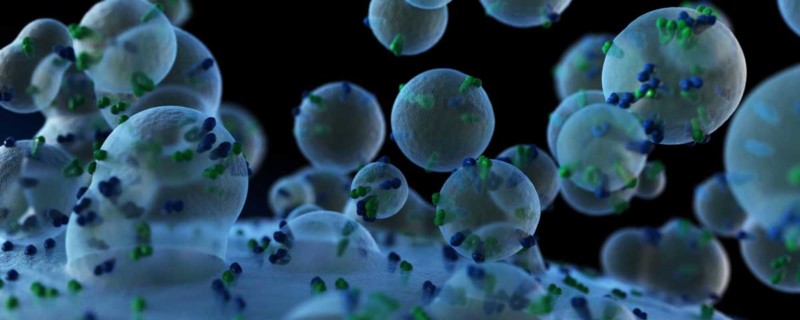
Manufacturing Acellular Regenerative Medicines
Challenges and opportunities in today’s manufacturing approaches
Introduction
Cell-based therapies such as CAR T or stem-cell therapies have become well-understood and are appearing in clinical practice. Cell-originated small structures and large molecules are increasingly appearing in developing therapeutic platforms. They support a diverse array of medicinal entities, including what are termed cell-free or acellular regenerative medicines (ARM) or therapies.
While such products as aspirin and mAbs are literally “cell-free” medicines, the phrase “acellular medicine” generally has a special definition referent to medicinal products that are either 1) biomimetic macromolecules or of cell-based origin, 2) smaller-biomolecule medicinal products replacing previously cell-based activity, or 3) therapeutic materials from which cells have been removed. Examples of such products include:
- Membrane-bound particles such as macrovesicles, apoptotic bodies, microvesicles, and exosomes
- Non-membrane-bound particles such as exomeres and supermeres [1]
- Some cytokines previously associated with a cell therapy
- Extracellular matrix materials and larger bioconstructs
- Decellularized ex vivo bone, tendon and cartilage
Cell-culture based ARM include endogenous regenerative technologies and extracellular-vesicle based therapies. Production, isolation, and purification of these products is an evolving and dynamic pursuit requiring creative solutions in technology transfer, manufacturing processes, and production facilities.
Extracellular Vesicles
Extracellular Vesicles (EVs, including exosomes) are naturally phospholipid enclosed nanovesicles released by all cell types. They have diverse diagnostic and therapeutic potential as they can harbour multiple functionally active biological molecules such as lipids, oligonucleotides, proteins, and intra-vesicle small molecules− as well as present targeting features on their surface. EVs play a vital role in normal physiology and cellular communication, are stable in circulation, and have low immunogenicity. They have been reported to exist in nearly every bodily fluid, and have been implicated in numerous natural functions. They are growing in popularity as non-invasive diagnostic, prognostic, and therapeutic tools [2, 3].
Applications
Diagnostic and prognostic
- Salivary exosomes are being used as biomarkers of oral and even systemic disease.
- Liquid biopsy from circulating blood contain numerous particles available for analysis: cells, cell debris, naked DNA, and EVs
Therapeutic
- Natural exosomes, e.g., those from mesenchymal stem cells have therapeutic potential and are key in many FDA clinical trials [4].
- Engineered exosomes, e.g., Codiak’s exoASOTM-STAT6, an engineered, targeted exosome delivering antisense oligonucleotides to down-modulate STAT6 mRNA expression [5].
Cell Culture-Based Production
Many acellular therapeutic products employ in vitro cell culture in their production. For EV manufacturing, there is a challenge in ensuring that a transition from lab-scale processes to larger scale manufacturing modes could lead to an inferior response of the cell phenotype or other lack of performance. EV Manufacturing modes include adherent or suspension culture. The types of bioreactors employed in ARM manufacturing include rotating wall/vessel, stirred tank, direct perfusion, wave, rotatory bed, parallel plate, fixed bed, 3D fibrous scaffolds, and hollow fiber [6]. Analysis of the surface components, size and concentration of EVs can include a variety of instrumentation supporting diverse technologies [7].
Isolation and Purification
Purification of EVs is problematic for a number of reasons, as many preparations of exosomes present contaminants of EV-related size and density, and EVs of a diverse size, density, loading, and surface components/ligands. First, development-scale activities often do not distinguish a product from such active sub-populations as product-related entities or impurities. Second, efficient small-scale isolation techniques do not scale well, and technical-transfer changes can affect isolation efficiency and the complement of sub-populations. Work is ongoing to standardize isolation and purification, and in current large-scale manufacturing, ultracentrifugation, TFF, column chromatography, immune affinity, are the preferred choices.
Commercialized solutions are making large-scale purification process development easier. One such example used for initial purification of many nanoparticle types is the Cytiva Capto Core 700 resin. It’s engineered with a molecular size cut-off outer layer, and an activated porous core. EVs exceeding 700kDa cannot enter the core, while small, soluble impurities penetrate and bind to the inner octylamide ligands.
Scaling
Processes are developed on a small scale and moving into manufacturing facilities is challenging, often leading to late choices in large-scale manufacturing technologies through the tech-transfer process. Scaling out can be an opportunity to increase production rate without encountering the complexities of scaling-up.
Batch Processing
Batch processing is still the go-to approach for most manufacturing. They are reliable from a documentation point-of-view for a small-scale process. However, in order to address a larger patient base, producing sufficient API mass can be a challenge.
Continuous Processing
While significant improvements are accruing in batch processing technologies, there is a general inclination to move towards a relatively continuous process via perfusion, packed-bed and hollow fiber approaches [6].
Facility Flexibility
The dynamic arena of ARM includes new and emerging entity types, manufacturing platforms, production modes, equipment offerings, and detection/isolation/purification/finish design. This calls for flexibility in production environments. Modern facility design approaches support process flexibility, future-proofing, single-use and functional closure, digital capability, automation, and efficient process and personnel flow [8]. Considerations in multi-modal facility design include suite dimensions, equipment requirements, closed and/or automated processes, service requirements, materials storage, entrance/egress designs, and emerging environmental sustainability imperatives. Creative suggestions for multi-modal facilities are now managed by modern BIM technologies. They yield multi-dimensional models supporting the design of both the immediate desired flexibility in equipment and process flow as well as efficiency through the entire product lifecycle. Facilities can be designed as stick-built, modular, or “Pod”ular. Each approach has its advantages and disadvantages in terms of on-site construction time, cost and flexibility of changes after the facility is built.
Room Classification
An FDA IND is required for clinical trials and FDA drug-level approval for clinical use, and this dictates requirements in facility design. With several operations currently being highly manual, there is a greater risk for contamination even if operations are carried out inside Biosafety Cabinets (BSCs) which are considered Grade B in a Grade C background. The use of isolator technology and closed, automated equipment allows for a reduction in the required room classification. Each isolator or closed devise is custom designed to its application. Several closed unit operations can be included in a single, low-classification suite, and multiple open operations in a single isolator, ranging from cell culture to final product filling.
Results and Discussion
- Novel ARMs are being extensively researched as an alternative to cell based and small molecule therapies. Bringing these to patients entails considerations ranging from PD and technology transfer, to manufacturing scale and suite design strategies.
- Production, isolation, and purification of these products is an evolving and dynamic pursuit requiring manufacturing facilities providing enhanced flexibility.
- Premier architectural design and build organizations are addressing the unique aspects of flexible facility design to support such innovative technologies.
About the Authors
William Whitford, Life Science Strategic Solutions Leader, DPS Group
Bill Whitford is the Life Science Strategic Solutions Leader for DPS Group Strategic Consulting Team, supporting innovative therapeutic developments and biomanufacturing processes. An invited lecturer at international conferences, Bill has published over 400 articles, book chapters and patents in the bioproduction arena and enjoys such appointments as serving on the BioProcessing International Editorial Advisory Board. He has an h-index of 16 and an i10 index of 25. An article of his recently brought the International Society of Pharmaceutical Engineers (ISPE) the 2022 APEX Award for Publication Excellence in the Technical & Technology Writing category for his article “AI’s Promise for ATMPs” published in the November-December 2021 issue of Pharmaceutical Engineering®.
Ankur K. Shah, Lead Process Engineer, DPS Group
Mr. Ankur Shah, a Lead Process Engineer at DPS Group’s Framingham Office, has over 14 years of experience performing process design for biopharmaceutical, nuclear waste remediation, mineral processing, and petrochemical facilities. Mr. Shah’s expertise extends in executing scoping studies through construction in Process Engineering and Project Engineering roles.
Footnotes
-
1. Zhang, Q., Jeppesen, D.K., Higginbotham, J.N. et al. Supermeres are functional extracellular nanoparticles replete with disease biomarkers and therapeutic targets. Nat Cell Biol 23, 1240–1254 (2021).
-
2. Jennifer Phan, Priyadarsini Kumar, Dake Hao, Kewa Gao, Diana Farmer & Aijun Wang (2018) Engineering mesenchymal stem cells to improve their exosome efficacy and yield for cell-free therapy, Journal of Extracellular Vesicles, 7:1, file:///C:/Users/william.whitford/Documents/2022/4.%20Presentations/5.%20ESACT%20Lisbon/MSC%20Exosomes%20GREAT%20paper.pdf
-
3. Kodam, S. P., Ullah, M., Diagnostic and Therapeutic Potential of Extracellular Vesicles, Review Article, Technology in Cancer Research & Treatment Volume 20: 1-10, 2021
-
4. ClinicalTrials.gov data, 287 Studies found for exosomes.
-
5. Codiak to Present New Preclinical Data Demonstrating Potent Monotherapy Activity of Two Engineered Exosome Intravenous Candidates Targeting Macrophages.
-
6. Whitford, W., Guterstam, P., Exosome manufacturing status William Future Med. Chem. (2019) 11(10), 1225–1236 ISSN 1756-8919 12. fmc-2018-0417 (future-science.com)
-
7. Luigi, I., Colao, I., Corteling, R., Bracewell, D., Wall, I., Manufacturing Exosomes: A Promising Therapeutic Platform.
-
8. The Future of Biopharma, How to Create a Flexible and Efficient Biopharmaceutical Production Facility.