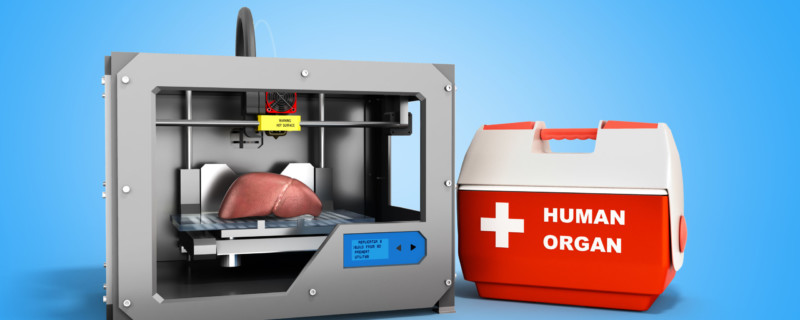
New Technologies to Facilitate 3D Bioprinting of Transplantable Organs
Broadly, the term “3D printing” refers to processes where a computer model of an object is recreated using materials are deposited and fused (such as polymer liquid or powder granules solidified together), layer by layer, to create a three-dimensional object. It is not surprising that this simple premise sparked innovation in other fields such as biomedical research. In this iteration, known as 3D bio-printing, living cells are deposited layer by layer in combination with biological scaffolds to form a desired 3D organic structure. While the spirit is the same as conventional 3D printing, bio-printing is considerably more complex because the functionality of tissues and organs is not solely based on physical structure. There are key interactions with other cells as well as their microenvironment, which inform their tissue-specific role. Nevertheless, this technique can be leveraged to fabricate complex, functional, cellular structures for many applications, including basic research, personalized medicine (disease modeling), regenerative medicine (transplantation or prosthetics) therapeutic investigation (drug screening/development) and for use in bioassays (cosmetics testing).
One of the most talked about topics, also hyped in the media, with regards to 3D bio-printing is its potential to artificially print whole, transplantable organs. The organ shortage is immense, with more than 113,000 individuals on the US national transplant waiting list as of July 2019. Advances in 3D bio-printing has led to an explosion in publications to address the challenges in building living tissue constructs in the shape of human organs.
Highlighted below are four innovative technologies developed this year by researchers to tackle some of the biggest problems in the quest to print 3D organs.
SLATE Technology
When looking at the complexities of an entire organ system, there is an interconnected network of blood vessels required to supply oxygen and nutrients to the tissues to maintain form and function. But, creating these intricate and invasive vascular networks in tissue engineering has been a hurdle until now.
Bioengineers Jordan Miller of Rice University and Kelly Stevens of the University of Washington (UW) pioneered a novel 3D bio-printing technology dubbed Stereo-Lithography Apparatus for Tissue Engineering (or SLATE for short) to print the vascular networks required by organs to fully function1. As a proof-of-concept, the researchers focused on recapitulating lung tissue. Using SLATE, they were able to print, layer by layer, a lung-mimicking structure complete with airways and blood vessels. The layers are generated using a liquid photopolymerizable pre-hydrogel solution that converts to a solid gel when exposed to blue light. The key breakthrough was the addition of non-toxic food dyes capable of absorbing blue light. These photo-absorbing molecules restrict the hydrogel solidification to a very fine layer allowing the production of soft, water-based, biocompatible gels with elaborate internal architecture. This detailed scaffold provided the architecture for cells to infiltrate post-fabrication to generate a functional network of vascular channels encapsulating the alveolar air sacs. Amazingly, when the vessels were mechanically challenged with artificial blood flow, they were strong enough to withstand the elastic movement caused by the “pulsating” blood and the rhythmic motions of inhaling and exhaling in the air sacs. Red blood cells moving through the vessels were also able to take up oxygen from the artificial air sac recapitulating the gas exchange that occurs in the human lung.
The stunning image of the 3D bio-printed lung structure, only the size of a penny, was featured on the cover of Science in May 2019. Miller and Grigoryan are commercializing key aspects of the research through a Houston-based startup company called Volumetric Bio.
Holographic Printing
Another group also tackling the task of printing complex vasculature is Prellis Biologics, founded in 2016 by Drs. Melanie Matheu and Noelle Mullin. The ultimate goal for the company is to produce fully transplantable 3D-printed organs, starting with kidneys. Their holographic 3D bio-printing technology is able to rapidly produce oxygen-supplying blood vessels and biocompatible tissue scaffolds. One key difference in their technology is that the living cells are part of the bio-ink, which is in contrast to other methodologies where cells are seeded post-printing onto the scaffolds. One of the biggest pain points is maintaining cell viability during the printing process. Traditional extrusion-based bio-printing cannot adequately address this problem, both because of the slow speed and lack of resolution. Prellis utilizes a laser-based technology where the bio-ink is combined with a light-sensitive photo-initiator, which can polymerize into a solid in under 5 milliseconds of exposure to infrared light. This rapid curing allows for printing of structures with an impressive level of resolution, 0.05 microns, a necessity to bio-print small vessels and tissue scaffolds.
At the end of July 2019, Prellis Biologics announced a total of $10.5 million in funding based on some exciting developments using this breakthrough technology to print intricate vascular networks2.
Vascular Tissue Blanks™ are one of Prellis Biologics product offerings and are ready-made, biocompatible 3D structures printed with capillaries that can be seeded with cells to facilitate rapid establishment of 3D models like organoids and larger tissue formats (3D Tissue Chip™, Organoids™, and the Vascular Bundle™). These blanks have been used with success on a variety of cell types including neurons, stem cells, vascular endothelial cells, primary human immune cells, and tumor cells for basic research, transplantation studies, drug screening/discovery and toxicological assays.
Prellis Biologics recently reported preliminary data from a transplantation study performed at Stanford University. Human tumors grown on vascular blanks and transplanted into mice were fully engrafted and vascularized using only 200,000 cells, a 10-fold decrease compared to numbers required for traditional tumor studies. Also, spontaneous growth of blood vessels from the animal’s own vasculature into the engrafted tumors was observed over an eight-week period. “A breakthrough like this opens the door to studying rare human tumors and complex human tumor immune system reactions,” explains Dr. Matheu. “It has the potential to significantly reduce overall animal use and speed up drug discovery efforts.” The details of the study will be made publicly available once it has been peer-reviewed.
The company continues to work on their goal of fully transplantable 3D-printed organs with work progressing to establish functionality of bio-printed human nephrons, the filtration unit of the human kidney. Animal testing of these structures is slated to begin in early 2020. As well, several other products are in beta test, one for establishment of biomaterial interfaces for toxicology screening and a second which acts as a human lung surrogate for studies requiring an air:liquid interface.
FRESH Technology
A group of researchers in Pennsylvania have published a new technology to overcome a common hurdle when 3D bio-printing with soft materials, like collagen. The resulting 3D structures are prone to sag and collapse without appropriate supports, limiting their success in recapitulating complex constructs. Scientists Andrew Lee, Andrew Hudson and collaborators from Carnegie Mellon University (CMU) have published their work on an advanced version of Freeform Reversible Embedding of Suspended Hydrogels (FRESH) technology, FRESH v2.0., to 3D bio-print small blood vessels, valves, and beating ventricles of the human heart with high fidelity and resolution3.
In the FRESH printing system, a bio-ink composed of an acidic collagen solution is extruded layer by layer into a neutral support gel (a mixture of gelatin microparticles and water), which can be removed post-fabrication once warmed to 37°C. The rapid change in pH experienced by the collagen drives its self-assembly while the gel provides structural support making it possible to 3D print with high resolution. The porous microstructure of the printed collagen also enables incorporation of human cells onto the fabricated scaffold. FRESH can also print with a wide range of other soft biomaterials including, but not limited to, alginate, fibrin, decellularized extracellular matrix, methacrylated gelatin, and methacrylated hyaluronic acid, widening the scope of this tissue engineering platform.
As described in Science, the group successfully bio-printed a full-sized tri-leaflet heart valve using collagen, which was able to maintain its mechanical integrity when tested with blood-like fluid flow velocities. As well, a model of the left ventricle of the heart was FRESH-printed using a dual-material printing methodology where collagen bio-ink, for structural integrity, was combined with a dense cellular bio-ink containing human embryonic stem cell-derived cardiomyocytes. The cardiomyocytes were sufficiently supported by the collagen scaffold to allow them to organize into the intended geometry in the ventricular model. Excitingly, the cardiac tissue showed spontaneous rhythmic contractions, directional action potential propagation and characteristic wall-thickening, all hallmarks of functional heart tissue. Finally, as a proof-of-concept, a neonatal-scale human heart was printed from collagen to demonstrate the potential of FRESH v2.0. at whole organ-scale while demonstrating high resolution.
FluidForm, a Massachusetts-based medical startup, is commercializing the FRESH technology (US patent 10,150,258) with its first product offering called LifeSupport™ bio-printing support gel to help researchers build advanced scaffolds for a wide range of tissues and organ systems.
SWIFT Technology
The previous technologies discussed have demonstrated exciting multi-material fabrication methods to generate 3D vasculature and tissues. However, these so-called “cells in gels” organ models have been found to have one to two orders of magnitude lower cell density than what is observed in vivo, and what would be required, to recapitulate physiological organ function4.
Now, a new technique called Sacrificial Writing Into Functional Tissue (SWIFT), created by Mark Skylar-Scott and Sébastien Uzel, researchers in Jennifer Lewis’ Lab from Harvard’s Wyss Institute for Biologically Inspired Engineering and John A. Paulson School of Engineering and Applied Sciences (SEAS), looks to overcome that major hurdle. Instead of trying to 3D print all of the cells in an entire organ, the group is focusing only on printing the blood vessels. Currently, 3D cellular models, such as embryoid bodies (EBs), organoids and multicellular spheroids exist for many tissue types and can recapitulate many organ-like functions but the lack of vasculature for nutrient and gas exchange limits their size, complexity and duration in culture. SWIFT looks to capitalize and improve upon these systems by printing the blood vessels directly onto tissue constructs created out of a network of these cellular aggregates, which they call stem-cell-derived organ building blocks (OBBs).
In the first stage, hundreds of stem-cell-derived aggregates (EBs, organoids, or spheroids) are formed into a matrix of OBBs. These aggregates are then compacted via centrifugation to form a densely packed OBB matrix. When this matrix is cooled (0-4°C), it is the perfect consistency to serve as a medium for sacrificial printing. During the second sacrificial printing stage, a thin nozzle “writes” gelatin ink throughout the matrix. Once the writing is complete, the matrix is warmed to 37°C where it takes on a more solid-like state while, at the same temperature, the gelatin ink melts away. This leaves behind a network of hollow channels interwoven throughout the OBB tissue construct of varying diameters – 400μm to 1mm. These channels allow oxygenated, nutrient-rich media to reach cells deep within the OBB matrix to support viable, organ-specific tissues with high cell density and function. When compared, tissue matrices SWIFT-printed with embedded vascular channels remained viable, while tissues grown in the absence of these channels suffered cell death in their cores within 12 hours.
To test the efficacy of the system to support organ-like function, the team created perfusable cardiac tissue containing human iPSC-derived cardiac OBBs. The perfused heart tissue maintained viability for over a week in culture and the cells contracted spontaneously and synchronously throughout the tissue, mimicking key features of a human heart. The SWIFT technology opens up new avenues to create personalized organ-specific tissues with embedded vascular channels for therapeutic applications.
Final Remarks
Certainly, these four innovative technologies are pushing the boundaries of the 3D bio-printing field. While the researchers are optimistic about these successes, they also recognize that many challenges still remain to print larger tissues on a commercial scale for ready-made, “off the shelf” formats – the huge number of cells required for these endeavors would be astronomical and poses a significant hurdle. Not to mention the regulatory landscape for clinical translation has yet to be defined. Nevertheless, it will be exciting to see where this research will lead as this nascent field progresses on the path to printing fully transplantable organs.
Footnotes
-
1. Grigoryan B., Paulsen S. J., Corbett D. C., Sazer D. W., Fortin C. L., Zaita A. J., Greenfield P. T., Calafat N. J., Gounley J. P., Ta A. H., Johansson F., Randles A., Rosenkrantz J. E., Louis-Rosenberg J. D., Galie P. A., Stevens K. R., Miller J. S. (2019). Multivascular networks and functional intravascular topologies within biocompatible hydrogels. Science364: 458–464. DOI: 10.1126/science.aav9750
-
2. Business Wire (July 30, 2019). Human Tissue Engineering Company Prellis Biologics Raises $8.7 Million Series A Round; Announces First Animal Transplant of Its 3D-Printed Tissue. Retrived from URL: https://www.businesswire.com/news/home/20190730005792/en/Human-Tissue-Engineering-Company-Prellis-Biologics-Raises
-
3. Lee A, Hudson AR, Shiwarski DJ, Tashman JW, Hinton TJ, Yerneni S, Bliley JM, Campbell PG, Feinberg AW (2019). 3D bio-printing of collagen to rebuild components of the human heart. Science, 365(6452):482-487. doi: 10.1126/science.aav9051
-
4. Skylar-Scott, M. A., Uzel, S., Nam, L. L., Ahrens, J. H., Truby, R. L., Damaraju, S., & Lewis, J. A. (2019). Biomanufacturing of organ-specific tissues with high cellular density and embedded vascular channels. Science advances, 5(9), eaaw2459. doi: 10.1126/sciadv.aaw2459