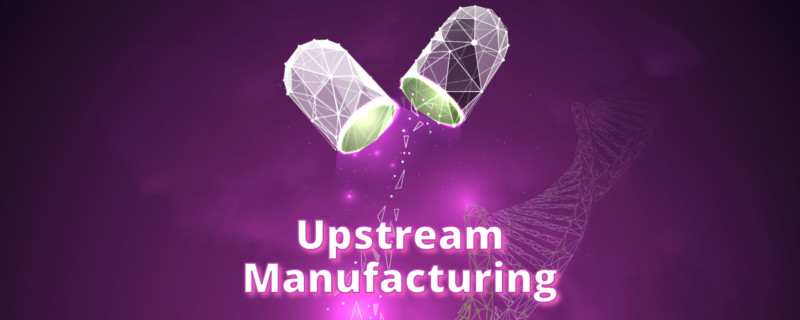
Upstream Manufacturing of Gene Therapy Viral Vectors
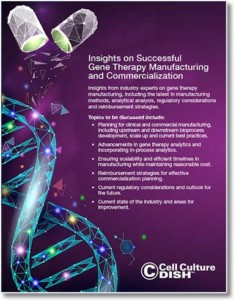
“Insights on Successful Gene Therapy Manufacturing and Commercialization”.
You can download all the articles in the series, by downloading the eBook.
Overview
The majority of gene therapy applications in development utilize viral vectors to carry the therapeutic gene into the target cells. Cells may be genetically modified either in vivo or ex vivo. In ex vivo applications, cells are modified in culture, which also allows for cell expansion and analytical characterization prior to re-infusion of the treated cells. This process has an advantage in that cells can be examined pre-treatment and post-treatment for viability, density, expression level, etc. The ex vivo process can sometimes result in more efficient transduction due to the greater control of the conditions. Alternatively, cellular modification by vectors can also be performed in vivo-directly in the subject. This approach avoids the need for cellular implantation.
There are several possible viral vectors systems available and the decision of which to use depends on many factors such as tissue tropism, desire for integrative or non-integrative modification, in vivo or ex vivo process, prior immune exposure, whether the target cell is replicating or non-replicating, safety, and others. Transduction at a high efficiency and robust levels of transgene expression are desired outcomes in viral vector applications.
A safety concern with viral vectors is generation of wild type infectious virus from vector components. For this reason, viral vectors are typically manufactured from 2, 3 or even several separate expressible units in order to significantly reduce the possibility of forming wild type virus via recombination. The units are typically separate plasmids introduced to producer cells either by transfection or through “helper” transducing viruses.
The vector units can be further engineered for safety by the placement of mutations or deletions, which would disable the function of the wild type virus should it be formed via a recombination event. For example, newer HIV lentiviral (LV) vectors delete genes for virulence factors tat, vpr, vpu, nef and/or have gag and pol on separate plasmids from rev and env1 and/or contain other mutations such as deletions in the 3’ LTR and some contain only a small percentage of complete HIV genome.2,3 Multi-plasmid transfection can also be favored for convenience, or by necessity in order to avoid toxicity of a vector component to producer cells (Figure 1).
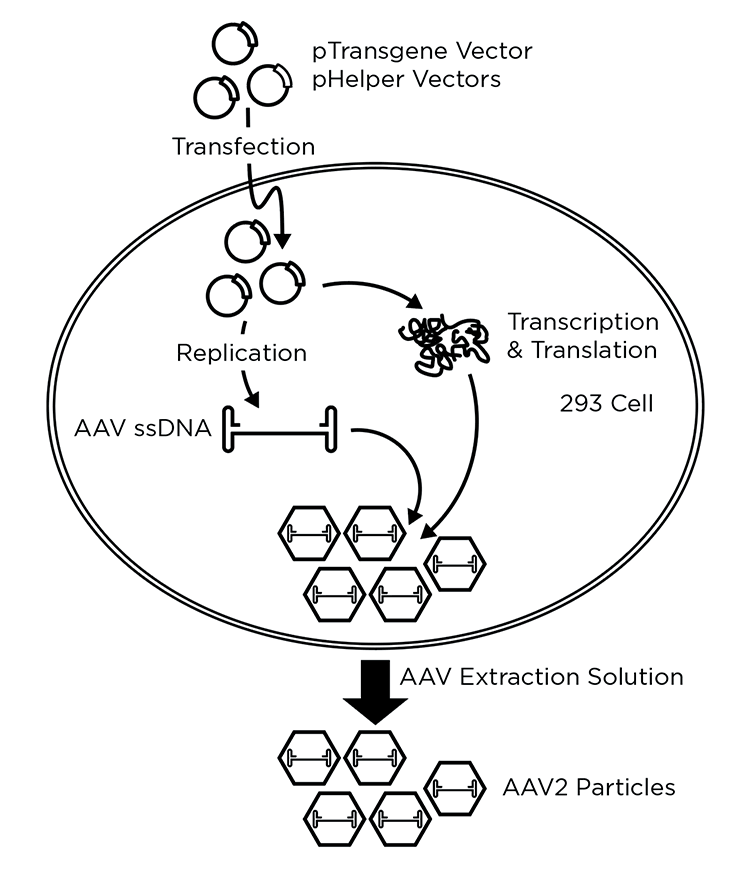
Major viral vectors in use
Retroviral (primarily gamma-retroviral) and adenoviral vectors were among the first cell modifying vectors and have generated a 30-year history to date.4 More recently, vectors derived from adeno-associated virus (AAV) and lentiviral (LV) vectors have advanced in development primarily due to enhanced safety and improved target tissue expression profiles. Other vectors such as herpes simplex virus and pox/vaccinia vectors, are entering the field and show promise in some applications such as oncolytic vaccines.
AAV is the most commonly used vector for in vivo genetic modification, and different serotypes (naturally occurring or recombinant) can be used to target different tissues within the body.5 AAV has been thought to be well tolerated, and results in a lower inflammatory response6,7, and generally thought to have a good safety profile. Moreover, unlike other viral vectors such as lentivirus that integrate into the host cell genome, AAV primarily remains episomal4,8 and has long term expression of the transgene.9
One possible limitation to the use of AAV is its lower transgene packaging capacity relative to other viruses at ~ 4.7 Kb (Table 1).6 This capacity limitation is not a major problem with many diseases being targeted and can sometimes be circumvented by minimizing promoter and ITR sequences or by the use of mini-genes of expression targets. Recent vector engineering efforts have demonstrated that AAV capacity can be increased to ~ 10 Kb by the use of split vectors to exploit the fact that AAV genomes form head-to–tail concatemers through homologous recombination within cells.9,10 Thus, by splitting the transgene between two vectors, the capacity of AAV can be roughly doubled-with current tradeoffs of reduced transgene expression and possible expression of unwanted products from reverse concatemerization.9,10 However, this work is still in experimental form.
Another challenge is that AAV can have a propensity to package any DNA in the vicinity, which may include host cell DNA and plasmid DNA.11,12 Other challenges can include lower transduction in humans than animal models, and the current methods of purification are somewhat immature. Nevertheless, AAV vectors have proven their potential and there is an ongoing effort to improve these vectors further through engineering.13
There have been some recent setbacks to the AAV platform. In 2020, three patients died in the high dose arm of the clinical trial of Audentes Therapeutics’s AT132, an AAV8 vector that delivers a copy of the myotubularin 1 gene.14,15 A few other patients in the higher dose arm receiving the higher dose also experienced adverse events. This event indicates that a clearer understanding is needed of the dosing limitations of an AAV vector. Nevertheless, despite these limitations and recent setbacks, AAV vectors show great promise for treatment of a wide variety of diseases, and there are a several AAV based therapies that have been approved worldwide.9,13 As a growing platform for gene therapy, AAV based therapeutics have a promising future.
Lentiviral vectors have gained much use recently, particularly in ex vivo applications, and are advantageous due to their ability to transduce both dividing and non-dividing cells. Current LV vector are primarily based on HIV-1, a virus that have been intensively characterized over the past years.
LV vectors have been key in the advancement of chimeric antigen receptor (CAR) T cell therapeutics which involve the transduction of primarily CD19 T cells, and CAR T therapies generated by retroviral vectors have been approved world wide as of 2019.13 LV transduction of slowly dividing CD34+ human stem cells has been applied to several genetic diseases, including ß-thalassemia16, X-linked adrenoleukodystrophy17, and metachromatic leukodystrophy.18
Another advantage to using LV vectors is that there are fewer challenges associated with insertional oncogenesis compared to gamma retroviral vectors (example MuLV), like those observed in the early clinical progress of gene therapies.1,19 LV vectors have advanced through 4 generations as of 2020 so that newer vectors contain as little as 4.8% sequence similarity to wild type HIV-120, which further advances their safety profile (for review see3).
The table below summarizes the physical properties of the four major viruses that are used for gene therapy:
Table 1. Overview of common viruses used for generating gene therapy viral vectors.
Parameter | Retrovirus | Lentivirus | AAV | Adenovirus |
---|---|---|---|---|
Coat | Enveloped | Enveloped | Non-Enveloped | Non-Enveloped |
Packaging capacity (Kb) | 8 | 8 | ~4.7(a) | 7.5 |
Tropism/infection | Dividing cells | Broad | Broad excluding hematopoietic stem cells | Broad |
Inflammatory potential | Reduced | Reduced | Reduced | High |
Host genome interaction | Integrating | Integrating | Integrating/ non-integrating | Non-integrating |
Transgene expression | Long-lasting | Long-lasting | Potentially long-lasting | Transient or long-lasting depending on immunogenicity |
Overview of viral vector manufacturing
The manufacture of viral vectors can be a complex process that may require several manufacturing phases or platforms. Initially, the materials needed to manufacture the therapeutic viral vector must be generated. These materials differ between vector types and design, but may include plasmids encoding helper-virus functions and the therapeutic gene, cell lines used to manufacture the vector, and others (See Figure 2, Left, generation of plasmids). In some cases, helper transducing viruses may be substituted for plasmids, which also need to be generated via cells. There are established packaging cell lines for some vector systems, such as gamma-retrovirus, while others are in development. One advantage to the use of stable lines for packaging is that they can reduce or eliminate transfection and/or transduction steps, simplify the production process, and lower costs.
The next step in vector manufacturing involves the generation of viral vector (Figure 2, Middle). Often cells are transfected with plasmids to generate the viral vector, which is harvested. Harvested vector is then concentrated, purified, titrated, characterized, and stored for later ex vivo or in vivo use. In the case of AAV and a few other vectors, viral particles may accumulate in both in the cytoplasm and the media. In that case, lysing the cells may enhance the total yield of vector.
If ex vivo transduction is being used (Figure 2, Right), target cells are collected and modified by the viral vector in a clean room. Following modification, the cells are harvested, characterized, and formulated prior to transplantation. In some processes, transduced cells may be expanded in cell culture prior to the infusion into patients.
Generation of viral vectors by transfection or infection
In general, there are two modes of vector production. The first is through a transient production system. Transient production systems involve either 1) transfection of one or several plasmids encoding the helper virus functions or 2) viruses to provide helper function.21 The second major mode of vector production uses a stable producer cell line. Stable producer lines do not require transfection or transduction to enable vector packaging, however, they do require substantial effort to generate. At the present time, the majority of vectors are currently generated by transient production systems due to convenience, or because of the lack of a stable producer cell line, or for other reasons.
Manufacturing viral vectors by transfection offers advantages and disadvantages. It is flexible and efficient, does not require time-consuming development of stable cell lines, and allows for successful vector generation should any viral component produce cellular toxicity upon expression.22 Producers that have a need to get to market quickly may favor manufacture by transfection because of its faster approach.
Transfection based methods do have disadvantages due to the requirement for costly GMP grade plasmids and the possible requirement of additional purification steps to remove DNA such as benzonase treatment. Currently there are many producers that produce plasmid, however, only a few offer GMP plasmid at scales required for large manufacturing. Moreover, transfection of suspension cells at larger scale can prove tricky to implement. Transfection can require time to optimize at any scale, and inconsistences between production batches is a concern in a manufacturing setting.
Key Manufacturing Platforms
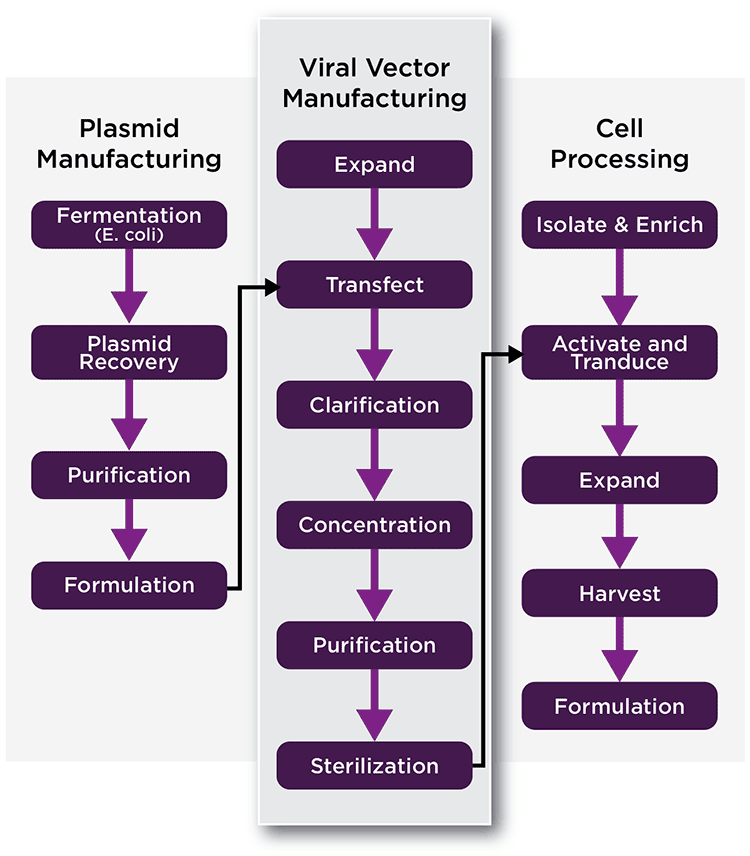
Among the transfection methods, polyethylenimine (PEI) transfection has advantages over calcium phosphate. It is generally less toxic to cells than other methods and the use of PEI can eliminate the need for a media exchange. PEI transfection is also less dependent on pH, the presence of serum, and is applicable for both adherent and suspension cultures.23 Drawbacks, however, include the large amount of costly plasmid required for large suspension culture and the absence of an analytical method to quantify PEI in the purified vector preparation.24 Lipid-based transfection reagents are effective but their cost may make them impractical for use at production scales.25 Non-chemical methods such as electroporation show promise, however, the need to concentrate cells can also make its use impractical at larger scales.24 Thus, transfection-based methods, while fast and efficient, can present significant challenges which include development time and concerns about consistency, particularly at larger manufacturing scales.
Use of baculoviral vectors for therapy or to produce other viral vectors
Low toxicity and the inability of baculoviruses to replicate in mammalian cells make them interesting candidates for therapeutic gene delivery.26 Baculovirus-mediated gene delivery into dividing and non-dividing mammalian cells has demonstrated therapeutic efficacy in both ex vivo and in vivo gene therapy studies.27-29
A suitable expression cassette and/or a pseudotyped envelope such as VSV-G can be used to transduce various cell types.30,31 Baculoviral vectors can mediate high-level transient expression of transgenes in many stem cells32 and can be modified to permit stable transgene expression.33 The high-level of transgene expression from baculoviral vectors is well suited for cancer gene therapy29,34 and the lack of pre-existing antibodies to baculovirus in humans is an advantage for in vivo therapy.
Baculoviral vectors have advantages and disadvantages. Baculoviral vectors offer an alternative to transfection for vector generation and have been used in the production of vectors such as AAV from insect cells35 and LV vectors from suspension cells.36 Baculovirus and other infection-based systems for the generation of vector are typically easier to work with at large scale. There are potential drawbacks to the use of baculoviral systems in that additional manufacturing steps are required in order to produce the baculoviral vector and remove it during downstream processing. Moreover, some cell culture media formulations can result in reduced transduction efficiency.27 Also, post-translational modification of viruses produced in insect cells can sometimes result in a reduction of potency. Overall, there is much promise for the use of baculoviral vectors and their use is growing in gene therapy vector development.
Stable producer cell lines
Cellular toxicity from the expression of a required vector component has complicated the establishment of stable producer cell lines for many vector systems. Toxicity from LV components (pro, pol, rev) has thwarted the development of stable LV producer cell lines. In the past few years, methods have been established for generating clinical-grade cell lines that continuously produce LV vector however many of these approaches currently lack desired robustness.37,38
Some stable producer lines utilize inducible promoters to minimize toxicity, and most stable MuLV retroviral cell lines are inducible.21 One example of cellular toxicity produced from a vector component is the pseudotyped retroviral envelope VSV-G1, which is can be substituted with an alternate envelope to improve toxicity.37 Toxicity is one aspect. Another is the prospect of making infectious virus without the transgene packaged. That effectively becomes an impurity that must be cleared via the purification process.
While vectors produced by transient expression of vector components continue to dominate the field, there has been a notable increase in the past couple of years in the use of stable production lines so that they now comprise about 30% of the products entering manufacturing.39 While the productivity of producer cells are currently no greater than transfection based methods39, their use can simplify manufacturing steps, lessen regulatory oversight, and lower cost. Thus, there can be significant advantages in the use of a stable producer cell line for therapies with large therapeutic demand or for those requiring high titers of vector.
Choice of producer cell line
Many factors influence the choice of cell line for vector generation. A key factor is production titer. Increased production titers of therapeutic vector can greatly reduce production scale and lower costs. For vectors produced by transfection, transfection efficiency is a major factor. HEK293, HeLa, and HT1080 cells generally have good transfection efficiency and can also produce high titers of vector.21,40 Among the many HEK lines, HEK293T cells reportedly produce increased titer because of the presence of SV40 large T-antigen.1 More recently “Fast” HEK293FT cells, which reportedly have shorter doubling times and greater production have been developed.41 Thus, many companies are presenting commercial cell lines with reportedly favorable properties, although there is always room for future improvement, such as with cell line engineering.
Note that the FDA suggests that HEK293T cells should not be used because of present technical and safety considerations with the presence of the SV40 T-antigen42. In addition, the FDA has undertaken recent studies looking at the feasibility of the use of T-antigen deleted HEK293T clones as a substitute for production of LV and AAV vectors.43
According to current US Food and Drug Administration (FDA) guidance for human gene therapy investigational new drug applications, if cells with a tumorigenic phenotype, such as HEK293T cells, are used for viral vector production, residual substrate DNA must be limited to assure product safety. In addition to controlling host cell DNA content; the level of transforming sequences in clinical products should be tightly controlled to limit patient exposure. Clinical products produced in HEK293T cells will require testing for residual adenovirus E1 and SV40 large T-antigen sequences.43,44
Scaling of adherent cells for manufacturing vector
The majority of the cell lines used to generate viral vectors are naturally adherent, with the exception of some tumor cell or blood cell lines which can grow in suspension.21 For adherent cells, the surface area of the culture device limits cell expansion. Hence, production scaling is accomplished either by increasing the number of identical culture systems units (flasks, roller bottle, cubes, HYPERStacks®) (scale out) or using successively larger devices (scale-up).45,46 In a manufacturing setting problems arise if production is scaled out since the number of increasing culture units can become unmanageable. Thus, scale-up into even larger units, such as bioreactors, can become an attractive option for increasing production scale.
Vector manufacturing has used “flatware” unit production systems of adherent based processes for some small-scale needs or for smaller clinical samples. These have mostly involved multilayer cell factories (CF) or HYPERFlasks®/Stacks (HY) (Corning). Cell factories have been used for the production of preclinical and clinical vector batches of ß-retroviral, lentiviral47 or AAV vectors.48 CFs can provide up to 2.5 m2 per unit, which is an increase over a roller bottle with area up to 0.17 m2.
Roller bottle expansion can be assisted by automation, which is commonly used in the vaccine industry; however, this requires significant utilization of specialized manufacturing facilities that limits feasibility. Cell factories are more difficult to scale than roller bottles and limitations in gas exchange in some conditions have been shown to decrease viral titer for AAV production.49 Some cell factories systems are available in semi-closed loops, providing some advantage.50
HYPERFlasks and HYPERStacks have a membrane for gas exchange which has been shown to increase LV production.51 These are more flexible than cell factories in media/surface area volumes and they offer areas of up to 1.8 m2 /unit. However, these also require the addition of more units for a significant increase in production scale. The Corning CellCube® system can scale-up to 34 m2. However, the CellCube system is only partly single-use.21
The use of bioreactors has advantages to increase production scale in that they uncomplicate increasing production scale by the use of fewer, larger vessels. Other advantages include easier monitoring and control of processes, reduced record keeping, fewer unit operations, lower contamination risks, reduced facility space, and lower operation costs.21,52,53 The improved control of culture condition in bioreactors may also result in improved productivity. Furthermore, many bioreactor systems can also be used in a perfusion mode which can be attractive for some vector systems such retroviral and lentiviral.21
Hollow fiber bioreactors, such as the Quantum® (Terumo BCT) utilize hollow fibers to provide an adherent surface for cells54, thus providing increased production scale in a small footprint.55 Fixed-bed bioreactor technology has been widely accepted in the industry. For example, the iCELLis® fixed-bed bioreactor (Pall Biotech) is used in the production of Zolgensma®, an AAV-based viral vector gene therapy used in the treatment of spinal muscular atrophy. The iCELLis bioreactor family currently consists of two models. The iCELLis Nano bioreactor is a benchtop unit used for process development and clinical production of virus at small scale. When larger scales are required, processes developed in the iCELLis Nano bioreactor can be easily scaled-up to the iCELLis 500+ bioreactor (Figure 3), the manufacturing-scale model.
The fixed-bed of the iCELLis bioreactor consists of polyethylene terephthalate (PET) macrocarriers (13.9 cm2 each) fixed inside a housing where media flows from the bottom to the top. The height of the bed can be adjusted as well as the compaction density of carrier. This enables a wide range of scalability options up to 500 m2 (Table 2). At maximum scale the iCELLis offers area that is roughly equivalent to 5900 roller bottles, 780 10-layer stacks, or 280 HYPERStacks (Table 2). In addition, the iCELLis bioreactor enables high cell density growth of 300,000 cells/cm2 and produces similar cell-specific titers compared to adherent culture.56 The iCELLis ™bioreactor is also provided on an industrial automation platform, easing the integration of this technology into existing manufacturing IT infrastructure.
Pall Biotech recently reported iCELLis performance and usability, cell distribution, pH, dissolved oxygen (DO), and metabolite profiles compared to flatware controls.57 The tests demonstrated a well-controlled system with homogeneous cell distribution. Pall Biotech has also demonstrated seed train usability through benchtop to industrial scale. Thus, these studies indicate that the iCELLis fixed bed systems provide confidence in moving rapidly from initial vector development to commercial scale production. These systems are suitable for the production of cell and gene therapy viral and vectors as well as for viruses used in vaccines.
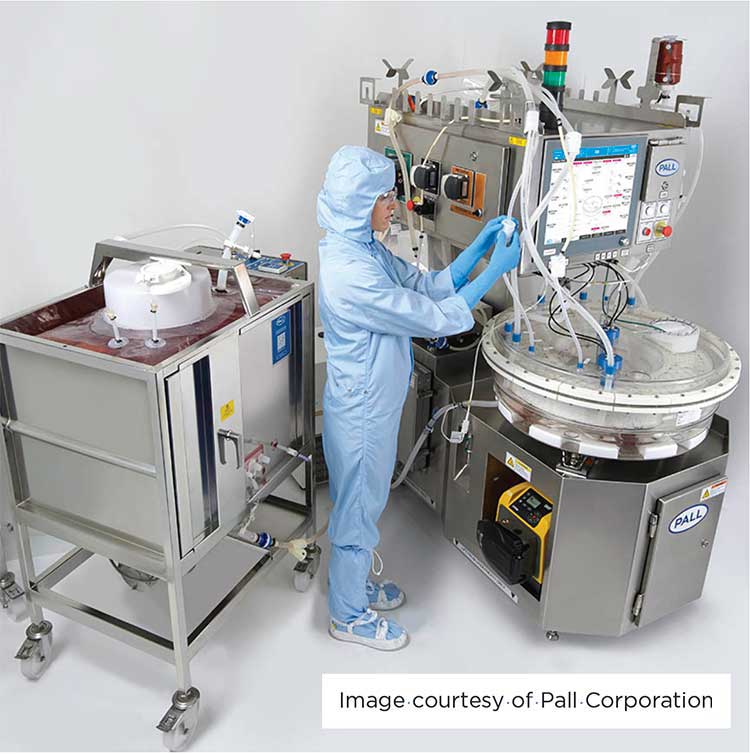
With the cell lines typically used for gene therapy production, the iCELLis at the 500 m2 scale has roughly the capacity of a 500L-1000L suspension cell, stirred tank, bioreactor. Thus, the iCELLis fixed bed reactor is an attractive enabling technology for therapeutics that require large or flexible production scale. This is particularly enabling for producers seeking a rapid time to market (producing a suspension cell line can take a year or more time) or for cell systems that are limited to adherent cells.
The cost benefits of the iCELLis bioreactor over multi-stack culture systems has been demonstrated in a modeling study that estimates cost reduction in a 100L to 1000L scale out scenario. The study modeled a typical LV production campaign via a transfection-based method. The results estimated the iCELLis would generate a 50% reduction in cost of goods, a 1.6x to 1.9x reduction in cost of goods through optimization (perfusion and plasmid use), and a 3x increase in throughput with perfusion. Furthermore, process complexity was significantly reduced. Thus, the iCELLis bioreactor produces numerous benefits over traditional flatware, that include significant cost savings and a simplified process.
Table 2. Scalability options for the iCELLis Nano and 500+ bioreactors.
Bed Size | Surface Area/m2 | Equivalent Units | ||||
---|---|---|---|---|---|---|
Bioreactor | Bed Diameter (cm) | Bed Height (cm) | Carrier Compaction Low-High | 850-cm2 Roller Bottles | 10-Layer Cell Stacks | HYPERStack 36 |
iCELLis Nano | 11 | 2 | 0.53 - 0.8 | 6.2-9.4 | 0.04-0.08 | 0.3-0.4 |
iCELLis Nano | 11 | 4 | 1.06 - 1.6 | 12.4-18.8 | 0.2-0.5 | 0.6-0.9 |
iCELLis Nano | 11 | 10 | 2.65 - 4.0 | 31.8-47.1 | 25-Oct | 1.5-2.2 |
iCELLis 500+/100 | 86 | 2 | 66 - 100 | 776-1116 | 104-157 | 37-56 |
iCELLis 500+/200 | 86 | 4 | 133 - 200 | 1565-2353 | 209-314 | 74-111 |
iCELLis 500+/500 | 86 | 10 | 333 - 500 | 3918-5882 | 524-786 | 185-278 |
Rocking bioreactors with adherent cell/microcarrier or single cell suspension culture are a potential option to produce smaller quantities of vector for research or clinical studies. Currently, their capacity is limited to 500L. Rocking bioreactors are disposable and can also be used to provide cell seed for larger bioreactors. Microcarriers can be effective for some cell types; however, their use requires much optimization to provide consistency as far as microcarrier type and surface, and of cell attachment protocol. Moreover, the ability to transfect/transduce cells growing on a microcarrier needs to be understood early in development.
Stirred tank bioreactors for manufacture of vector
While hollow fiber and fixed-bed bioreactor systems offer significant increases in scale, the scale is currently limited compared to stirred tank bioreactors that can extend into the thousands of liters. Virus production in the vaccine industry has been successfully scaled up to more than 2000 liters using microcarriers in stirred tank bioreactors59 using adherent Vero or MRC5 cell lines. A 2000L bioreactor operating at 10 cm2/mL microcarrier density can theoretically offer 2,000 m2 of surface area, if the complex technical optimizations involving the use of microcarriers can be perfectly optimized and developed.
Stirred-tank bioreactors are by far the most prevalent bioreactor used for the commercial manufacturing of mAbs and recombinant proteins. As a result, the technology is very well characterized and both industry and regulatory authorities are very familiar with their operation. They are the most efficient means of scaling up to large volumes. While these bioreactors can be used to grow adherent cells with the use of microcarriers, they are best adapted to the growth of suspension cells. HEK293 mammalian cells as well as SF9 insect cells can be adapted to suspension culture.
Stirred tank bioreactors are offered in a variety of size and configuration options. For example, the Allegro™ STR bioreactor (Pall Biotech) is a single-use bioreactor that is available in 50, 200, 500, 1000 and 2000 L scales (Figure 4). In addition, the Allegro STR bioreactors have a compact footprint due to their cubical design, allowing for an installed height of less than 3 m even at the 2000 L scale. BrammerBio has shown that insect cell culture can be successfully carried out in the Allegro STR bioreactors, allowing for cell density up to 7 million cells/mL.60 The wide range of models offer flexibility of choice in production scale as well as a convenient path though cell seed generation through seed train.
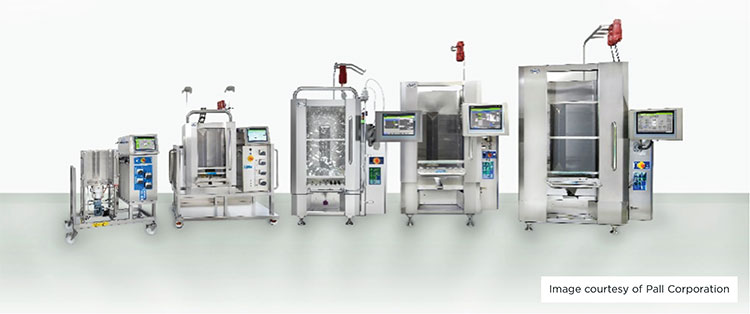
One of the important considerations in selecting bioreactors for up-scaling of production is performance as well as ease of use and compatibility through the sizes. Studies have shown linear scalability from Allegro STR 50L to 500L bioreactors for the production of AAV from HEK293 suspension cells and that there is no significant difference between the two bioreactors as long as the same process is performed (Figure 5).58 Moreover, cell doubling time was reduced in the Allegro STR 50 bioreactor (26 hours) compared to shake flasks (34 hours). This would be expected from the controlled conditions and improved monitoring.
Scaling of suspension cells for manufacturing vector
A major advantage of single-cell suspension culture over adherent cells is that they can easily be scaled up from spinner, to laboratory scale bioreactor, to production bioreactor without burdensome cell detachment steps. The use of animal free media is a desirable feature for manufacturing due to the decreased risk of adventitious agents and the reduced purification burden. Several HEK293 cell lines used for vector production (293T, 293FT) are prone to adaptation to suspension culture in chemically defined media61 and suspension HEK293 cell lines pre-adapted to commercial media are available on the market. Bauler et al. recently described the creation of a GMP compatible suspension HEK293T cell line that doubled LV production compared to flatware and a 10x increase in transducing units compared to two commercial systems.62 Published reports have shown that high titers of LV vector can be achieved using a suspension adapted helper cell line in small-scale bioreactors.62,63
Current challenges and limitations of suspension cell culture
It can take some time to adapt a custom cell line to high growth, up to a year and more, which can result in a delay of time to market for a therapeutic. Moreover, there is no guarantee that the adaptation effort will result in a suspension cell line with equal or greater cell-specific productivity than the originating adherent cells. In addition, there are currently challenges associated with transfection of cells at scales >200L which can present a limiting factor to the adoption of suspension culture for transfection dependent processes.24 Furthermore, extensive development time is usually required to optimize the efficiency of transfection at larger scale.
Another challenge is that many of the cell lines currently used in vector production do not necessarily achieve high single-cell density growth. For example, HEK293 cells can be prone to clumping at densities above 2 million cells/mL in current media formulations.39 Thus, it is important for the vector developer to understand the full advantages and disadvantages of the use of suspension culture for their particular vector, cell, and market. While the use of suspension cell lines currently remains a smaller fraction of the market, there has been a notable increase in their adoption in the past couple of years.39
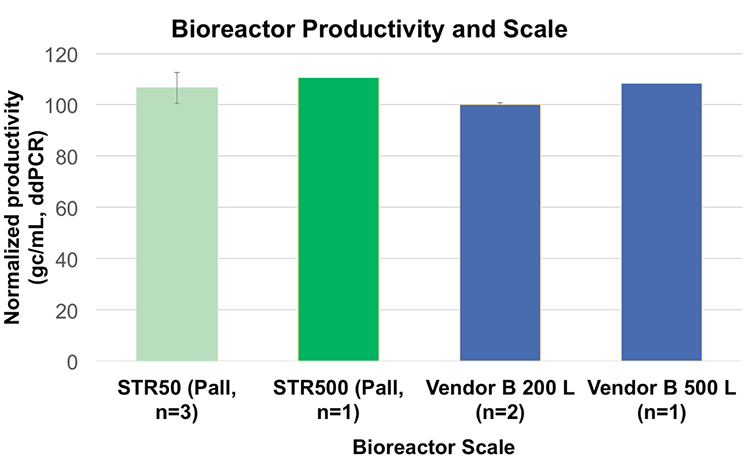
Cost considerations
Vector based gene therapies can be expensive, and up to $1-2 million/dose. It is to be expected that production costs will decrease in the future as new technologies advance in the emerging vector production industry. Currently, there are several factors that can influence the cost of viral based gene therapy manufacturing. These include production titer, the ability to up-scale production processes, regulatory burden, and other factors.
Production titer is a key factor as higher titer results in a smaller, more cost-effective production process. High titer production of vector can lower reagent demand, labor, and facility requirement, in addition to enabling a more efficient downstream operation. One possible route to increase titer is through control of the cell environment and of production conditions. In this regard, controlled bioreactor vessels can present an advantage over flasks, roller bottles, CFs, or other flatware in productivity.
In addition, use of bioreactors can simplify scale-up of a process as previously described. From a capital expenditure, point of view, scaling up multilayer stacks requires considerable space. Since clean room space is expensive, this can be a very significant cost. In contrast, implementation of a bioreactor system can be done in the same space required for a modestly size multilayer stack system.
Application of a bioreactor system can decrease labor and consumables costs 3-fold and there is considerably less plastic waste generated with a bioreactor system resulting in reduced disposal costs. Most bioreactor systems come with some degree of automation, which manifests itself as a 3-fold decrease in labor, compared to more manual systems like multilayer stacks. A greater than 50% reduction in costs can be achieved by implementing a bioreactor system compared to a multilayer stack of similar scale.64 Scaling out a process that uses adherent cells in multilayer CF requires many connections and incubators, so contamination risk increases along with capital expenditure costs. Finally, disposable systems like the iCELLis bioreactor, or other single use systems can provide savings as cleaning, sterilization as well as extensive validations are reduced or eliminated. The use of bioreactors also results in lower risk due to the reduction of production units, especially compared to a scale-out, multi-production unit configuration.
Other expenses include screening for producer cells and vector batches for replication-competent viruses and other regulatory requirements.65 Limitations of vector sterilization may also influence costs given that large viruses, such as HSV, may not be filter sterilizable, thus requiring aseptic processing, and validation that goes with that. As some gene therapy products are relatively new for regulatory authorities, it is highly likely that regulatory guidance will increase as more products are taken to market, which may add to future costs.66
Process design and regulatory burden as a cost determinant
Production design can have a large effect on cost. While vector production by transfection is easy, and flexible, and quick to market, it increases in costs and presents technical challenges as scale increases. The cost of GMP plasmid can be one of the largest cost drivers of upstream operations.
The use of helper viruses (for direct infection) rather than plasmids may reduce transfection needs and provide a less expensive path for scaling up production. In this regard, the use of a stable producer cell line to reduce or eliminate transfections and transductions can be of great benefit through reduced manufacturing steps, which lower costs.
The use of a stable producer cell line over transfection methods may also facilitate process consistency, which is an attribute for GMP vector production.65 Since all components of vector production intended for therapy require GMP manufacture, optimization or elimination of costly GMP steps is an important consideration.67
Meeting regulatory specifications is critical requirement. Optimization of the overall process design in light of regulatory burden for purity and safety can greatly lower production costs.66 These include the presence of potentially toxic or immunogenic impurities, contaminants such as HCP proteins and DNA, plasmid DNA, reagents from transfection, potential transduction inhibitors, induction agents, antibiotics, and others.68 Other optimizations include optimizing transfection to minimize costly plasmid consumption, optimizing cell culture medium feed to improve productivity, and optimizing cell harvest from large bioreactors so that they are pooled to enter downstream processing in a single path.
While there is significant work in optimizing the overall process there are significant long-term benefits. Overall, it has been estimated a non-optimized production process, like those used in academia, can increase costs 40% and can reduce dose throughput 33%.39
Process development timeline considerations
Required speed to market and overall costs are two considerations of process development. Adherent based bioreactor systems such as the iCELLis bioreactor can have advantages over suspension cell systems in terms of process development timelines. There is less required cell development and optimization can move relatively fast and adaptation of an adherent cell process from cell factories can be relatively short. By contrast, adapting an adherent cell system to suspension cell system can be a very lengthy process (8-18 months)-which can delay the time of product to market.
Transfection based approaches often enter production because they are inherited from academic development of the vector. Transfection based processes generally are quick and flexible and can move at a faster pace into market. However, they are expensive, and may induce technical and cost difficulties at larger scale required for some viral vectors. Many producers are currently entering market using transfection and adherent cells because it is fast and provides a fast route to market.
For products that require high yield, lower costs, and have a longer allowed time for introduction to market, undergoing development of suspension cells or a stable producer line can have long term benefit, even though it can take months or years to develop either. Indeed, some vector producers choose to enter market using a transfection/adherent cell process while concurrently developing stable producer cells, suspension cells, or both for future use.
Process development must be done smartly with defined goals in mind. As such it can take considerable time to develop a process that meets strict regulatory standards. There are many parts to an upstream process such as media optimization, cell line optimization, creation of cell banks and use of (stable) cell lines. All of these require considerable consideration and time to be successful in the market.
Analytical considerations
The final vector product intended for gene therapy and vaccine applications must be well characterized and of proper quantity, purity and potency for both producers and regulatory authorities. This can be achieved only if adequate characterization methods are in place and the viral production process is well established, understood and reproducible. Control and product characterization are not only important for the final product, but also within the different steps of the production process. In depth knowledge of both up- and downstream processing is crucial since the upstream production greatly affects the downstream process. Sometimes even slight changes in the upstream process can result in a less efficient downstream process.
There has been continued advancement of analytics for vector production. The well-established framework for traditional biologics (i.e. recombinant proteins, enzyme replacement therapies, monoclonal antibodies) is increasingly being applied to the field of gene therapies. As such, even more investment in real-time, high-throughput technologies to characterize both process and product intermediates is needed for vector manufacturing.
Impact of upstream on downstream purification
Many vectors are secreted into the media. In this case, virus can be collected from the media, which will substantially reduce downstream burden. With some vectors, a portion of the virus remains in the cells and so cell lysis with either detergent or physical means may be critical to improve yield. There are a wide variety of processes that can be used for purification, including clarification by filtration or centrifugation; chromatography steps for affinity, ion exchange, sizing, multi-modal and other resins; concentration steps with TFF or ultracentrifugation; and enzymatic treatments to reduce nucleic acids. When the cells must be lysed to release the vector products, multiple steps may be needed to optimize the purification.
The choice of upstream production process can affect downstream purification burden. For example, shear forces on adherent cells on microcarrier systems or other systems with fluid motion can increase cell debris. Another example is that a fixed-bed bioreactor can offer some benefit since some impurities can be trapped into the bed. For downstream production engineers, the greatest concerns are the productivity, quality and consistency of material that arrives from upstream operations.
There has been increased interest and work in the area on how vector design and upstream process can impact final vector product infectivity and quality – and lessen purification burden. Two aspects of quality of a vector preparation are the levels of 1) full particles to empty particles and 2) the level of infective capsids to non-infective particles. These essentially determine the potency of the product and potentially the levels of side effects, such as loss of potency or immune (CTL) response.69 Theoretically, a perfect vector preparation would have a total particle (vp) to infectious particle (iu) ratio of 1.70 It has been noted that highly purified preparations of wild type AAV2, for example can contain nearly this ratio.70 However, identically purified rAAV vector preps can have an infectivity of less than one out of 100 particles.70 Thus, there is room for improvement in vector design, cell lines, or methods for the production of recombinant vectors.
It has been noted that several factors can influence these quality parameters, such as culture methods (shake flasks vs fed batch flask vs bioreactor)71, production via transfection vs transduction71,72, and others. It has also been noted that the relative distribution of AAV particles in cell lysate and culture media can be dependent on the particular vector.73 Thus, there is much to learn and improve for the production of vector with high specific productivity.
Room for Improvement
There are several areas of improvements for viral vectors for gene therapy. One of the current challenges in the market is to understand the product and how the production process can impact the product. There is generally good knowledge of both the individual virus and production processes. However, there is not much current experience with the interplay of the two in producing a viral product. This is an emerging industry and there is much to learn as it moves forward.
As mentioned earlier, transfection can be both problematic at larger scale and expensive to produces doses required for some clinical applications. More advancement in the use of stable producer lines is needed. The development of stable producer cell lines that are also adapted to suspension may further increase production by enabling the use of large tank bioreactors. These, in turn, can be used in closed systems with less risk of contamination and the use of serum-free, animal-free media will lessen purification burden and improve regulatory safety profiles.
The cost of vector and vector-derived therapies continue to present a challenge towards advancement. Methods need to be developed which result in lower costs similar to what has occurred in the monoclonal antibody industry.
Current methods for lysing cells (example AAV) are also crude and there is room for improvement. Alkylphenol ethoxylate (APE) surfactants such as nonylphenol ethoxylates (NPEs) and octylphenol ethoxylates (OPEs), have restrictions on use in the EU and will be severely restricted in Jan. 2021.74 This restriction will include Triton X-100, which is has been one of the most widely used lysing agents.
Despite the ongoing need for improvements in therapeutic vector production, there is much optimism for the future of viral based gene therapy. In the future, vector based gene therapy may not only offer gene replacement, but could advance to offer gene editing functions as well.
Conclusion and recent shifts in upstream processing
One of the most notable shifts in the past 2-3 years has been the approval of numerous vector based therapies by regulatory authorities. This serves as validation of this new, emerging industry and it is a significant move forward. In addition, there is more experience and recognition of the production scales needed for some vectors, such as AAV, to go to market.
Over the past 5 years, production has gradually shifted towards suspension cells, a trend which appears to have accelerated in the past 12 months.75 Just a few years ago, nearly 100% of products were produced via transfection of adherent cells. At the present time, adherent cells are used in roughly 70% of products in development39, including two FDA approved gene therapies. The shift to suspension cells requires developmental effort that is ongoing and although there are currently no approved products that use baculovirus vectors13, there has been a notable increase in their development as well.
While there is a perception that cost of goods would be lower for a suspension process, this may not be true currently. On average these cell lines currently produce less virus than their ad-herent counterparts, which in turn would increase the cost per dose both in terms of cell line and plasmid raw material requirements.75
There has also been a notable increase in the use of stable producer cells, particularly for the production of LV vectors.39 LV vectors to date have been primarily utilized for ex vivo CAR T therapies, which do not require large titers of virus and is well suited for production via transfection. The use of stable cell lines and the advancement of LV vectors could potentially assist the expansion of LV therapies into in vivo applications.
There has been more recognition by the industry that the molecular biology of the cell, the expression system, and the vector matter for production yield- or for clinical therapeutic properties. It is entirely possible that we are at the early stages of the re-engineering of systems so that they are designed for improved outcome. On the production side there is more work being performed on suspension cells, which in turn, would assist large production scale. There is also discussion of cell clone selection or cellular modification to increase vector productivity at the cellular level. It is entirely possible that these approaches are being imported from the monoclonal antibody industry.
On the clinical side, there is ongoing discussion about AAV capsid design to increase specificity toward target tissues. Improved tissue targeting would result in lower dosing of vector, which in turn would reduce manufacturing burden, lower cost, and improve safety. Lower dosing could also enable therapies that would otherwise require prohibitively high titers of vector. Thus, there is more work being done on the molecular biology of gene therapy vectors and the cells that produce them.
There has been a continued shift to the use of fixed bed bioreactors over conventional flatware culture for adherent cells. Fixed bed bioreactors, such as the iCELLis bioreactor, provide a route to increased scale of production up to 500L and a quicker route to market.39 Their use has been enabling for the industry as they can and move production away from an unmanageable number of flatware vessels into a single bioreactor.
There are also advancements in medium scale transfection methods up to 60-70L. There is greater understanding of the large-scale transfection process as gentle mixing and delivering DNA rapidly to the cell within a few minutes are critical parameters.39 New pump and mixing technology has allowed transfection at some increased scale, without a loss of productivity.39 Challenges remain for larger multi-hundred liter scale.
In summary, we see continued advancement and growth in an emerging industry that now has several approved therapeutics on the market and a bright future.
Footnotes
-
1. Milone, M. C. & O'Doherty, U. Clinical use of lentiviral vectors. Leukemia 32, 1529-1541 (2018).
-
2. Dull, T. et al. A third-generation lentivirus vector with a conditional packaging system. J Virol 72, 8463-8471 (1998).
-
3. Galeh, H., Bolandian, M., Dorostkar, R. & Jafari, A. Concise review on optimized methods in production and transduction of lentiviral vector in order to facilitate immunotherapy and gene therapy. Biomedicine Pharm 128, 1-11 (2020).
-
4. Hastie, E. & Samulski, R. J. Adeno-associated virus at 50: a golden anniversary of discovery, research, and gene therapy success--a personal perspective. Hum Gene Ther 26, 257-265 (2015).
-
5. Balakrishnan, B. & Jayandharan, G. R. Basic biology of adeno-associated virus (AAV) vectors used in gene therapy. Curr Gene Ther 14, 86-100 (2014).
-
6. Colella, P., Ronzitti, G. & Mingozzi, F. Emerging issues in AAV-mediated In vivo gene therapy. Mol Ther Methods Clin Dev 8, 87-104 (2018).
-
7. Merten, O. W. AAV vector production: state of the art developments and remaining challenges. Cell GeneTherapy Insights 2, 521-551 (2016).
-
8. Srivastava, A. Advances and challenges in the use of recombinant adeno-associated virus vectors for human gene therapy. Cell GeneTherapy Insights 2, 553-575 (2016).
-
9. Li, C. & Samulski, R. J. Engineering adeno-associated virus vectors for gene therapy. Nature reviews. Genetics 21, 255-272 (2020).
-
10. Li, C., Bowles, D. E., van Dyke, T. & Samulski, R. J. Adeno-associated virus vectors: potential applications for cancer gene therapy. Cancer Gene Ther 12, 913-925 (2005).
-
11. Chadeuf, G., Ciron, C., Moullier, P. & Salvetti, A. Evidence for encapsidation of prokaryotic sequences during recombinant adeno-associated virus production and their in vivo persistence after vector delivery. Mol Ther 12, 744-753 (2005).
-
12. Schnodt, M. et al. DNA minicircle technology improves purity of adeno-associated viral vector preparations. Mol Ther Nucleic Acids 5, e355 (2016).
-
13. Ma, C., Wang, Z.-L., Xu, T., Z-Y, H. & Wei, Y.-Q. Approved gene therapy drugs worldwide: from 1998 to 2009. Biotechnol Adv 40, 1-13 (2020).
-
14. Dearment, A. (Aug. 21, 2020). Astellas’ Audentes Therapeutics discloses third patient death in gene therapy trial. Accessed Retrieved from https://medcitynews.com/2020/08/astellas-audentes-therapeutics-discloses-third-patient-death-in-gene-therapy-trial/
-
15. Taylor, N. Astellas' Audentes reports 3rd death in gene therapy trial. Accessed Retrieved from https://www.fiercebiotech.com/biotech/astellas-audentes-reports-third-death-gene-therapy-trial
-
16. Cavazzana-Calvo, M. et al. Transfusion independence and HMGA2 activation after gene therapy of human beta-thalassaemia. Nature 467, 318-322 (2010).
-
17. Cartier, N. et al. Hematopoietic stem cell gene therapy with a lentiviral vector in X-linked adrenoleukodystrophy. Science 326, 818-823 (2009).
-
18. Biffi, A. et al. Lentiviral hematopoietic stem cell gene therapy benefits metachromatic leukodystrophy. Science 341, 1233158 (2013).
-
19. Hematti, P. et al. Distinct genomic integration of MLV and SIV vectors in primate hematopoietic stem and progenitor cells. PLoS Biol 2, e423 (2004).
-
20. Vink, C. A. et al. Eliminating HIV-1 Packaging Sequences from Lentiviral Vector Proviruses Enhances Safety and Expedites Gene Transfer for Gene Therapy. Mol Ther 25, 1790-1804 (2017).
-
21. Merten, O. W., Schweizer, M., Chahal, P. & Kamen, A. Manufacturing of viral vectors for gene therapy: part I. Upstream processing. Pharm. Bioprocess. 2, 182-203 (2014).
-
22. Krieg, A. M. Direct immunologic activities of CpG DNA and implications for gene therapy. J Gene Med 1, 56-63 (1999).
-
23. Segura, M. M., Garnier, A., Durocher, Y., Ansorge, S. & Kamen, A. New protocol for lentiviral vector mass production. Methods Mol Biol 614, 39-52 (2010).
-
24. Merten, O. W., Hebben, M. & Bovolenta, C. Production of lentiviral vectors. Mol Ther Methods Clin Dev 3, 16017 (2016).
-
25. Cribbs, A. P., Kennedy, A., Gregory, B. & Brennan, F. M. Simplified production and concentration of lentiviral vectors to achieve high transduction in primary human T cells. BMC Biotechnol 13, 98 (2013).
-
26. Kost, T. A., Condreay, J. P. & Jarvis, D. L. Baculovirus as versatile vectors for protein expression in insect and mammalian cells. Nat Biotechnol 23, 567-575 (2005).
-
27. Mahonen, A. J. et al. Culture medium induced vimentin reorganization associates with enhanced baculovirus-mediated gene delivery. J Biotechnol 145, 111-119 (2010).
-
28. Madhan, S., Prabakaran, M. & Kwang, J. Baculovirus as vaccine vectors. Curr Gene Ther 10, 201-213 (2010).
-
29. Wang, S. & Balasundaram, G. Potential cancer gene therapy by baculoviral transduction. Curr Gene Ther 10, 214-225 (2010).
-
30. Volkman, L. E. & Goldsmith, P. A. In vitro survey of Autographa californica nuclear polyhedrosis virus interaction with nontarget vertebrate host cells. Appl Environ Microbiol 45, 1085-1093 (1983).
-
31. Tani, H. et al. In vitro and in vivo gene delivery by recombinant baculoviruses. J Virol 77, 9799-9808 (2003).
-
32. Ho, Y. C., Chung, Y. C., Hwang, S. M., Wang, K. C. & Hu, Y. C. Transgene expression and differentiation of baculovirus-transduced human mesenchymal stem cells. J Gene Med 7, 860-868 (2005).
-
33. Ramachandra, C. J. et al. Efficient recombinase-mediated cassette exchange at the AAVS1 locus in human embryonic stem cells using baculoviral vectors. Nucleic Acids Res 39, e107 (2011).
-
34. Luo, W. Y. et al. Development of the hybrid Sleeping Beauty: baculovirus vector for sustained gene expression and cancer therapy. Gene Ther 19, 844-851 (2012).
-
35. Urabe, M., Ding, C. & Kotin, R. M. Insect cells as a factory to produce adeno-associated virus type 2 vectors. Hum Gene Ther 13, 1935-1943 (2002).
-
36. Lesch, H. P. et al. Production and purification of lentiviral vectors generated in 293T suspension cells with baculoviral vectors. Gene Ther 18, 531-538 (2011).
-
37. Park, J. et al. Progressing from transient to stable packaging cell lines for continuous production of lentiviral and gammaretroviral vectors. Curr Opin Chem Eng 22, 128-137 (2018).
-
38. Sanber, K. S. et al. Construction of stable packaging cell lines for clinical lentiviral vector production. Sci Rep 5, 9021 (2015).
-
39. Pall Biotech. Current observation.
-
40. Gama-Norton, L. et al. Lentivirus production is influenced by SV40 large T-antigen and chromosomal integration of the vector in HEK293 cells Hum Gene Ther 10, 1269-1279 (2011).
-
41. Thermo Fisher. Accessed Retrieved from https://www.thermofisher.com/order/catalog/product/R70007
-
42. National Inst. for Innovation in Manufacturing Biopharmaceuticals (NIIMBL) (Nov. 2018). Gene Therapy Roadmap. Accessed Oct. 10, 2020: Retrieved from https://niimbl.force.com/s/gene-therapy-roadmap
-
43. Bae, D. H. et al. Design and Testing of Vector-Producing HEK293T Cells Bearing a Genomic Deletion of the SV40 T Antigen Coding Region. Mol Ther Methods Clin Dev 18, 631-638 (2020).
-
44. US FDA. Chemistry, manufacturing, and control (CMC) information for human gene therapy investigational new drug applications (INDs)-Guidance for industry. Accessed Retrieved from https://www.fda.gov/regulatory-information/search-fda-guidance-documents/chemistry-manufacturing-and-control-cmc-information-human-gene-therapy-investigational-new-drug
-
45. Segura, M. M., Mangion, M., Gaillet, B. & Garnier, A. New developments in lentiviral vector design, production and purification. Expert Opin Biol Ther 13, 987-1011 (2013)
-
46. Spier, R. in Animal Cell Biotechnology, Vol. 1 (eds Spier, RE & Griffiths, JB) (Academic Press, 1985).
-
47. Merten, O. W. et al. Large-scale manufacture and characterization of a lentiviral vector produced for clinical ex vivo gene therapy application. Hum Gene Ther 22, 343-356 (2011).
-
48. Allay, J. A. et al. Good manufacturing practice production of self-complementary serotype 8 adeno-associated viral vector for a hemophilia B clinical trial. Hum Gene Ther 22, 595-604 (2011).
-
49. Okada, T. et al. Large-scale production of recombinant viruses by use of a large culture vessel with active gassing. Hum Gene Ther 16, 1212-1218 (2005).
-
50. Pakos, V. & Johansson, A. Large scale production of human fibroblast interferon in multitray battery systems. Dev Biol Stand 60, 317-320 (1985).
-
51. Kutner, R. H., Puthli, S., Marino, M. P. & Reiser, J. Simplified production and concentration of HIV-1-based lentiviral vectors using HYPERFlask vessels and anion exchange membrane chromatography. BMC Biotechnol 9, 10 (2009).
-
52. Ghani, K., Cottin, S., Kamen, A. & Caruso, M. Generation of a high-titer packaging cell line for the production of retroviral vectors in suspension and serum-free media. Gene Ther 14, 1705-1711 (2007).
-
53. Merten, O. W. State-of-the-art of the production of retroviral vectors. J Gene Med 6 Suppl 1, S105-124 (2004).
-
54. Sheu, J. et al. Large-scale production of lentiviral vector in a closed system hollow fiber bioreactor. Mol Ther Methods Clin Dev 2, 15020 (2015).
-
55. Lennaertz, A., Knowles, S., Drugmand, J.-C. & Castillo, J. Viral vector production in the integrity® iCELLis® single-use fixed-bed bioreactor, from bench-scale to industrial scale. BMC Proc. 7, 59 (2013).
-
56. Lennaertz, A., Knowles, S., Drugmand, J.-C. & Castillo, J. Viral vector production in the integrity® iCELLis® single-use fixed-bed bioreactor, from bench-scale to industrial scale. BMC Proc. 7, 59 (2013).
-
57. Pall Biotech. Application Note: iCEllis 500+ single-use Q series 333 m2 bioreactor cell culture validation study.
-
58. Gene therapy company (name not disclosed). Internal data.
-
59. Barrett, P. N., Mundt, W., Kistner, O. & Howard, M. K. Vero cell platform in vaccine production: moving towards cell culture-based viral vaccines. Expert Rev Vaccines 8, 607-618 (2009).
-
60. Snyder, R., Glover, C. & Vaidya, R. Accelerating The Development of Viral Vector Manufacturing Processes. Accessed Retrieved from https://www.pharmasalmanac.com/articles/accelerating-the-development-of-viral-vector-manufacturing-processes
-
61. Dormond, E. et al. An efficient and scalable process for helper-dependent adenoviral vector production using polyethylenimine-adenofection. Biotechnol Bioeng 102, 800-810 (2009).
-
62. Bauler, M. et al. Production of Lentiviral Vectors Using Suspension Cells Grown in Serum-free Media. Mol Ther Methods Clin Dev 17, 58-68 (2020).
-
63. Manceur, A. P. et al. Scalable lentiviral vector production using stable HEK293SF producer cell lines. Hum Gene Ther Methods 28, 330-339 (2017).
-
64. Pall Biotech. Cost modeling of upstream production process of lentiviral vectors in HEK-293 cells comparing multi-tray 10 stacks and fixed-bed bioreactor. Poster ESACT 2017 (2017).
-
65. McCarron, A., Donnelley, M. & Parsons, D. Scale-up of lentiviral vectors for gene therapy: advances and challenges. Cell GeneTherapy Insights 3, 719-729 (2017).
-
66. White, M., Whittaker, R., Gandara, C. & Stoll, E. A. A guide to approaching regulatory considerations for lentiviral-mediated gene therapies. Hum Gene Ther Methods 28, 163-176 (2017).
-
67. Ausubel, L. J. et al. Production of CGMP-Grade Lentiviral Vectors. Bioprocess Int 10, 32-43 (2012).
-
68. Rodrigues, T., Carrondo, M. J., Alves, P. M. & Cruz, P. E. Purification of retroviral vectors for clinical application: biological implications and technological challenges. J Biotechnol 127, 520-541 (2007).
-
69. Gao, K. et al. Empty virions In AAV8 vector preparations reduce transduction efficiency and may cause total viral particle dose-limiting side-efffects. Mol Ther Methods Clin Dev 1, 20139 (2014).
-
70. Zeltner, N., Kohlbrenner, E., Clément, N., Weber, T. & Linden, R. M. Near-perfect infectivity of wild-type AAV as benchmark for infectivity of recombinant AAV vectors. Gene Ther 17, 872-879 (2010).
-
71. Joshi, P. R. H. et al. Achieving high-yield production of functional AAV5 gene delivery vectors via fedbatch in an insect Cell-One baculovirus system. Mol Ther Methods Clin Dev 13, 279-289 (2019).
-
72. Adamson-Small, L. et al. A scalable method for the production of high-titer and high-quality adeno-associated type 9 vectors using the HSV platform. Mol Ther Methods Clin Dev 3, 16031 (2016).
-
73. Piras, B. A. et al. Distribution of AAV8 particles in cell lysates and culture media changes with time and is dependent on the recombinant vector. Mol Ther Methods Clin Dev 3, 16015 (2016).
-
74. CRS. CRS-Chemical inspection and regulation service -REACH Restriction. Accessed Retrieved from http://www.cirs-reach.com/REACH/REACH_Restriction.html
-
75. Cameau, E. & Capone, J. Evolution of culture production systems for viral vector production: advantages, challenges and cost considerations. Cell Gene Ther. Insights 6, 225-230 (2020).