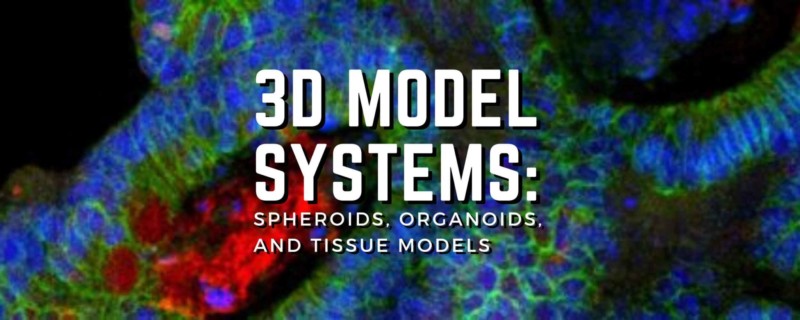
3D Model Systems: Spheroids, Organoid and Tissue Model Systems
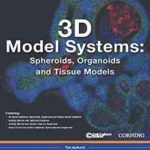
“3D Model Systems: Spheroids, Organoids and Tissue Models”.
You can download all the articles in the series, by downloading the eBook.
Culturing cells outside of their natural environment in a laboratory under controlled conditions has become essential to scientific research. Cell culture has utility in diverse areas from stem cell and cancer research, monoclonal antibody production, drug discovery, regenerative medicine, therapeutic protein production and for modeling diseases. Cells to establish in vitro cultures can be isolated from normal or diseased tissues, be grown as adherent monolayers or in suspension, and can be established in two or three dimensions.
With the methodology established over a century ago, traditional two-dimensional (2D) tissue culture involves cells proliferating on flat substrates such as glass or polystyrene, resulting in monolayer cell cultures. However, this is a far departure from how cells grow in vivo, lacking critical cell-cell and cell-matrix interactions in their native microenvironment that drive their form, function and response to external stimuli. This has driven the development of more realistic and predictive three-dimensional (3D) cell culture models to improve the prognostic capability of in vitro testing systems. Culturing cells in 3D more accurately recapitulates the in vivo state where the cell morphology, interactions and tissue-specific architecture more closely resembles that of native tissues.
The terms spheroid and organoid are commonly used when anyone refers to 3D cell culture systems. Spheroids and organoids in the most basic sense are both 3D structures made of many cells, so what’s the difference? It can be particularly confusing since this terminology has been used interchangeably in the literature. Here, we look to demystify these two terms and discuss the distinct differences between them and their utility in scientific research as well as more advanced 3D tissue model systems like organ-on-a-chip technology.
Spheroid
The term ‘spheroid’ was first coined in the 1970s by Sutherland et al1 when it was discovered that dissociated Chinese hamster V79 lung cells were able to form almost perfectly spherical cellular aggregates in suspension when grown in spinner flasks. Since then, spheroids have been generated from many primary cells and cell lines in a multitude of ways from hanging drop plates, low cell attachment plates, micropatterned surfaces or rotating bioreactors to promote aggregation. Most commonly, the term spheroid is used in cancer research where tumor cells constitute the classical multicellular tumor spheroid model (MCTS), which has been invaluable to the study of solid tumor biology. The spherical geometry of the cells within the MCTS are characterized by an external proliferating region and an internal quiescent zone (caused by the gradient of nutrient and oxygen diffusion), which surrounds a necrotic core, mimicking the cellular heterogeneity observed in solid tumors. The unique cytoarchitecture of cells within the MCTS emulate cell morphology, proliferation, oxygenation, nutrient uptake, waste excretion and drug uptake. As such, the MCTS model is the most widely used preclinical screening tool for anticancer drug candidates2.
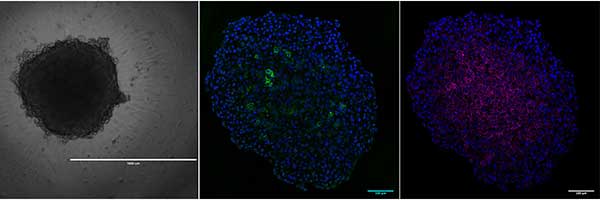
That said, the absence of strict terminology has resulted in confusion surrounding what constitutes a spheroid. For example, the term “organoid”, now defined as “mini-organs”, has been used in the past to describe 3D structure spontaneously formed by colon carcinoma cell line LIM1863, for 3D cultures of tumor cells embedded in basement membrane–like gel, and even the MCTS2.
Organoids
Organoid is a general term that has been around for many years. In the past, it was defined as an aggregation of cells that contained differentiated cells with some tissue-like structures3, which is what we know now as spheroids. In the recent resurgence of the organoid, they are now classified as a 3D structure grown from stem cells or organ progenitors and consisting of organ-specific cell types that self-organizes through cell sorting and spatially restricted lineage commitment mimicking at least one function of tissue or organ4.
Organoids (Figure 2) can be classified into those that are tissue-derived and those that are stem cell-derived. Tissue-derived organoids typically originate from adult tissues while stem cell-derived organoids are established from pluripotent stem cells. As with their in vivo counterparts, organoids contain multiple cell types organized in structures that resemble the organ of interest and exhibit some of the organ function. Organoids representing different organ systems have been generated, such as the brain, liver, thymus, thyroid, lung, pancreas, intestines and heart5. These models have been used to study organ development, perform drug discovery/screening, model diseases, for toxicity testing and as therapeutic tools.
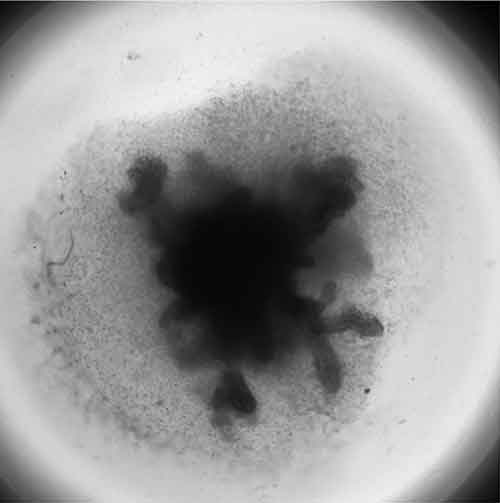
Generally, a higher order of self-assembly in found in organoids compared to spheroid cultures, which are more simplistic, possessing little or no relevant tissue structures. The formation of organoids is more dependent on the presence of biological or synthetic matrices. Interaction between the cell and the extracellular matrix (ECM) is essential for survival, proliferation, differentiation and migration6. The ECM also provides a physical structure upon which cells can move and grow in 3-D. Spheroid cultures are not as dependent on matrices for their formation, able to form in scaffold-free and scaffold-based conditions7.
The reasons for pursuing 3D cell culture models is clear: it is simply a better way to grow cells in a way that most closely resembles how they grow and interact with each other and their microenvironment in native tissues. Indeed, it is widely accepted that organoids and spheroids are superior to 2D monolayers but technical challenges and costs has hampered their adoption into high throughput screening protocols8. Current methodologies are bogged down by low experimental throughput, standardization issues and in some cases, poor reproducibility. Additionally, thorough validation/characterization of these models against standard 2D assays and animal models is required before they can be widely adopted for drug discovery protocols8,9. That said, the wealth of knowledge grows daily and advances in 3D printing and technology platforms will made it easier, faster and cheaper to put these platforms in place for large-format, high throughput protocols.
3D Tissue Model Systems
While spheroid and organoid 3D cultures are a marked improvement over monolayer cultures, they still fail to mimic the full architecture of in vivo tissues, which includes vasculature and interstitial fluid flow. Emerging ‘organ-on-a-chip’ (OOC) technology may be able to address some of these limitations. By definition, OOC are flexible polymer devices representing the convergence of microfluidics and tissue engineering10. Cells are cultured in continuously perfused micrometer-sized chambers, which creates physiologically relevant levels of of fluidic sheer force and provides nutrition, gas and waste transport to replicate in vivo vascularized tissues (Figure 3). The first of these was the lung-on-a-chip11 designed by the Wyss Institute at Harvard University, which was used to study the effect of bacterial infection on lung function. Since then, tissue model systems have been designed for heart12, kidney13, artery14, bone15, cartilage16, skin17 and the female reproductive tract18.
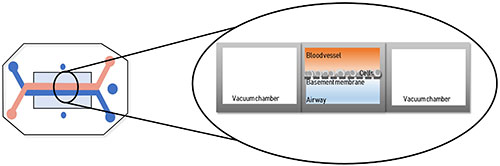
The higher degree of complexity and similarity of OOCs to native tissues makes them excellent tools to elucidate organ-specific biomechanical and biochemical mechanisms and for use in drug discovery programs. This platform has the potential to decrease cost, replace animal testing and streamline drug discovery workflows to identify drug candidates that are safe and efficacious in humans. Ultimately, the goal would a ‘human-on-a-chip’ system, where multiple organs are connected to assess the “whole body” response, to replace drug screening in cell culture and animal models. While the ‘organ-on-a-chip’ technology shows great promise, we are a long way from reaching that goal. They still lack many of the complexities observed in real tissues, therefore, more research is needed for them to become effective catalysts for drug discovery.
Footnotes
-
1. Inch W.R., McCredie J.A., Sutherland R.M. Growth of nodular carcinomas in rodents compared with multi-cell spheroids in tissue culture (1970). Growth. 34:271–282.
-
2. Weiswald LB, Bellet D, Dangles-Marie V. Spherical cancer models in tumor biology (2015). Neoplasia. 17(1):1–15. doi:10.1016/j.neo.2014.12.004
-
3. Clevers, H. Modeling Development and Disease with Organoids (2016). Cell. 165 (7):1586-1597. doi: 10.1016/j.cell.2016.05.082
-
4. Lancaster MA, Knoblich JA. Organogenesis in a dish: modeling development and disease using organoid technologies (2014). Science. 345(6194):1247125. doi: 10.1126/science.1247125
-
5. Drost J, Clevers H. Organoids in cancer research (2018). Nat Can Reviews. 18 (7):407-418. doi: 10.1038/s41568-018-0007-6
-
6. Li Y, Xu C, Ma T. In vitro organogenesis from pluripotent stem cells (2014). Organogenesis. 10(2):159–163. doi:10.4161/org.28918
-
7. Villasante A, Vunjak-Novakovic G. Tissue-engineered models of human tumors for cancer research (2015). Expert Opin Drug Discov. 10(3):257–268. doi:10.1517/17460441.2015.1009442
-
8. Nees M and Åkerfelt M. Beyond ‘simple’ biology – turning organoids, spheroids, and 3D tissue models into physiologically relevant high-content assays for drug discovery. Drug Target Review, 11 December 2018, https://www.drugtargetreview.com/article/38041/high-content-assays-for-drug-discovery/2/ Accessed April 13, 2019
-
9. Simeonov A, Lal-Nag M. High-throughput screening platforms incorporating physiologically relevant 3-D models. Drug Target Review, 8 January 2017, https://www.drugtargetreview.com/article/36595/high-throughput-screening-platforms-incorporating-physiologically-relevant-3-d-models/ Accessed April 13, 2019.
-
10. Bhatia SN, Ingber DE. Microfluidic organs-on-chips (2014). Nat. Biotechol. 32(8):760-72. doi: 10.1038/nbt.2989.
-
11. Huh D, Matthews BD, Mammoto A, et al. Reconstituting organ‐level lung functions on a chip (2010). Science. 328:1662–1668.
-
12. Watson, D. E., Hunziker, R., & Wikswo, J. P. Fitting tissue chips and microphysiological systems into the grand scheme of medicine, biology, pharmacology, and toxicology (2017). Experimental biology and medicine (Maywood, N.J.). 242(16): 1559–1572. doi:10.1177/1535370217732765
-
13. Wilmer MJ, Ng CP, Lanz HL, Vulto P, Suter-Dick L, Masereeuw R. Kidney-on-a-Chip Technology for Drug-Induced Nephrotoxicity Screening (2016). Trends Biotechnol. 34: 156–170. doi:10.1016/j.tibtech.2015.11.001
-
14. Günther A, Yasotharan S, Vagaon A, et al. A microfluidic platform for probing small artery structure and function (2010). Lab Chip.10(18):2341–2349. doi:10.1039/c004675b.
-
15. Torisawa Y, Spina CS, Mammoto T., Mammoto A., Weaver JC, Tat T. Bone marrow-on-a-chip replicates hematopoietic niche physiology in vitro (2014). Nat Methods. 11:663–669.
-
16. Lin H, Cheng AW, Alexander PG, Beck AM, Tuan RS. Cartilage tissue engineering application of injectable gelatin hydrogel with in situ visible-light-activated gelation capability in both air and aqueous solution (2014). Tissue Eng Part A. 20 (17-18):2402–2411. doi:10.1089/ten.TEA.2013.0642
-
17. Wufuer M, Lee G, Hur W, et al. Skin-on-a-chip model simulating inflammation, edema and drug-based treatment (2016). Sci Rep. 6:37471. doi:10.1038/srep37471
-
18. Xiao S, Coppeta JR, Rogers HB et al. A microfluidic culture model of the human reproductive tract and 28-day menstrual cycle (2017). Nature Communications 8:14584