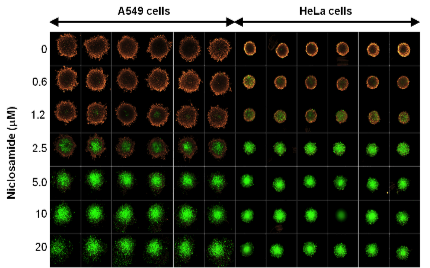
A New Dimension of Cell Culture: The Rise of Spheroid Culture Systems
In this article, we discuss the evolution of spheroid culture systems, methodologies of spheroid formation, spheroid culture challenges and relevant solutions.
Historically, much of the knowledge we have on cellular processes is from experiments where cells cultured on flat, two-dimensional (2D) surfaces. 2D cell culture systems span many applications from studying basic cellular processes, stem cell and cancer research to regenerative medicine. While these systems are attractive because they are simple, cheap, and work for a variety of cell types, there are some limitations. Cells cultured on planar surfaces do not reflect the in vivo physiology such as tissue-specific architecture, cell-to-cell and cell-to-matrix connections, which can affect their proliferation, function and response to external stimuli1. This is evidenced by the fact that 2D model systems are not always predictive, resulting in disappointingly high failure rates when used in new drug discovery and clinical trials1. Despite these disadvantages, 2D cell cultures are still routinely used because they are well-established and historical data based on 2D cultures allows researchers to execute comparative studies. Additionally, most of the equipment used for high throughput screening (HTS) is compatible with and optimized for 2D culture systems making them amenable to large scale screening protocols2.
More recently, three-dimensional (3D) cell culture systems are emerging as powerful tools with huge translational potential, particularly for cancer and stem cell research. 3D cell culture models are better able to recapitulate not only the in vivo morphology, but the cell connectivity, polarity, gene expression and tissue architecture, making them more physiologically relevant and predictive compared to 2D models; therefore, bridging the gap between in vitro and in vivo models1. That said, many 3D culture techniques are time-consuming and cumbersome making them difficult to incorporate into existing cell culture workflows. Nutrient and oxygen gradients along with diffusion kinetics are also factors to be considered when culturing cells in 3D especially as the size of the cellular aggregate grows4. Additionally, imaging and quantitative analysis of 3D cultures may also pose a challenge since most instruments and protocols are designed for 2D culture systems3. While the technology for generating 3D cell cultures have progressed over the past decade, there are still more innovations to come as researchers discover the potential of 3D tissue culture applications.
Spheroids
Multicellular spheroids are three-dimensional spherical cellular aggregates and one of the most common and versatile way to culture cells in 3D. Many cell types traditionally cultured in 2D have been adapted to grow in 3D spheroid systems, which has renewed their utility in many applications, particularly in cancer research and drug discovery. Some examples of spheroids include multicellular tumor spheroids (MCTS), neurospheres, mammospheres, hepatospheres and embryoid bodies1,4.
3D cell culture has long been used in oncology to study solid tumor biology. The most well-characterized is the MCTS model (Figure 1). Tumor spheroids have facilitated a better understanding of cancer biology and the development of more sensitive drug screening platforms due to their biosimilarity to solid tumors in vivo with regards to cell morphology, proliferation, oxygenation, nutrient uptake, waste excretion and drug uptake5,6. As such, the MCTS model has become an invaluable tool for testing cancer therapeutics since poor drug penetration to the hypoxic region within solid tumors is thought to be the cause of many cancer drug failures4.
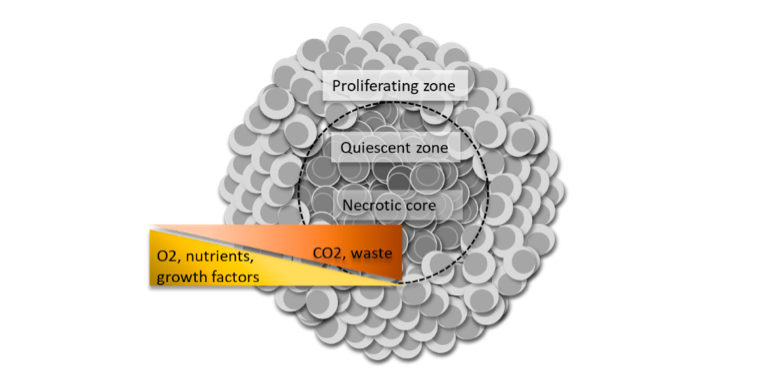
More recently, spheroid cultures have demonstrated their value in regenerative medicine. Mesenchymal stem cell (MSC) spheroid cultures were found to have greater anti-inflammatory and tissue regeneration and repair potential, as well as improved cell survival post-transplant compared to their 2D-cultured counterparts1,7.
Scaffold vs. Scaffold-free Methodologies for Spheroid Formation
The type of 3D cell culture technique chosen depends on the type of the cells, tissue origin (i.e. cell line, primary cell), the aim of the study and need for high throughput capabilities. There are a large number of commercially available culturing tools for 3D spheroid formation and can be separated into scaffold-based and scaffold-free techniques8.
Scaffold-based Techniques
The extracellular matrix (ECM) is an important component of the cellular microenvironment in native tissues where cell-to-matrix connections are integral for survival, proliferation, differentiation and migration of cells. Scaffolds aim to mimic the native ECM and can be composed of a variety of biological and synthetic materials with differing porosities, permeability, surface chemistries, and stiffness intended to mimic the microenvironment of specific tissues to promote cell growth and migration1,4. Examples of scaffold-based 3D culture methods include micropatterned plates9, microcarrier beads10 and more sophisticated microfluidics systems4,10,11,12. Both the composition and stiffness of the extracellular matrix surrounding the cells as well as biological properties such as cell compatibility or adhesiveness have major effects on cell signaling and behavior13,14.
Biological scaffolds such as collagen and Matrigel™ (derived from the Engelbreth-Holm-Swarm (EHS) mouse sarcoma cells) are commonly used for spheroid formation4. They not only provide physical support for cells to attach and reorganize into 3D structures, they can contribute soluble growth factors, hormones, and other unidentified components, which can affect gene and protein expression13,14. Therefore, researchers need to consider these matrix interactions when selecting a 3D scaffold for a particular application as these interactions can confound results.
Polymeric scaffolds use synthetic hydrogels or other biocompatible polymeric materials to generate the physical supports for 3D cultures. They can offer more design flexibility than biological scaffolds, modified to have desired ECM characteristics for the cell type of interest to encourage cell aggregation while maintaining tissue functionality4. These polymers are biologically inert, which circumvents problems that arise from using biological scaffolds, as mentioned above. Poly(ethylene glycol) (PEG) is commonly used for this application due to its nontoxicity and non-immunogenicity4. A major drawback of synthetic scaffolds is it can require skilled technician and extra time to execute tedious fabrication steps15.
In an effort to increase throughput, microfabrication to form concave hydrogel microwell arrays have shown potential to generate uniform-sized multicellular spheroids9. One such example is a poly(ethylene glycol)(PEG)-based hydrogel micropatterned plate, which enable the efficient generation of, as well as the culture of, uniform-sized embryoid bodies used for neural differentiation studies9. However, subsequent characterization and analysis of cell spheroids on such hydrogel arrays as well as mass production of the device present challenges to researchers and manufacturers alike.
Microfluidic platforms are a more sophisticated method, which adds an additional level of complexity by introducing a perfusion aspect to the culture environment10,14. Commonly referred to as “organ-on-a-chip”, these multi-channel systems replicate key functions of living organs allowing for continuous nutrition and oxygen introduction as well as waste removal, thus creating a more realistic and dynamic biologic environment8. Typically, the microchannels are coated or filled with hydrogel for trapping and providing a scaffold for cell growth10,11. This system also facilitates the generation of larger, more complex spheroids and also allows spheroids to be maintained for long-term studies since the parameters of their microenvironment can be tightly controlled11.
Scaffold-free Techniques
In contrast, scaffold-free spheroid methods do not use biomaterials or ECM components to support cell-to-cell attachment and migration. These techniques promote cell-to-cell adhesion (over cell-to-surface) as the key driver to their formation. In this forced self-assembly process, cells secrete their own ECM components, which more accurately recapitulates the in vivo events that occur during embryogenesis, morphogenesis and organogenesis10. Tumor cell biologists commonly uses scaffold-free methods to form tumor spheroids to study gene expression or drug sensitivity14.
Scaffold-free methods can be divided into agitation-based and agitation-free11. Rotating flasks and bioreactors are a relatively simple way to form spheroids through continuous stirring of the culture medium, which keeps cells in suspension to form aggregates1,10,15. The main drawback is that a broad size range of spheroids is generated and the shear stress from continuous agitation is undesirable. Agitation-free methods include the use of hanging drop plates (gravity forced aggregation)1,10,15, low cell attachment plates1,17, and emerging techniques like magnetic levitation18, and magnetic 3D bioprinting19. The advantages of scaffold-free methods are that they can be applied to many different cell types and are relatively easy to execute.
Figure 2 outlines some of the most common methods, both scaffold-based and scaffold-free, for 3D spheroid formation discussed here.
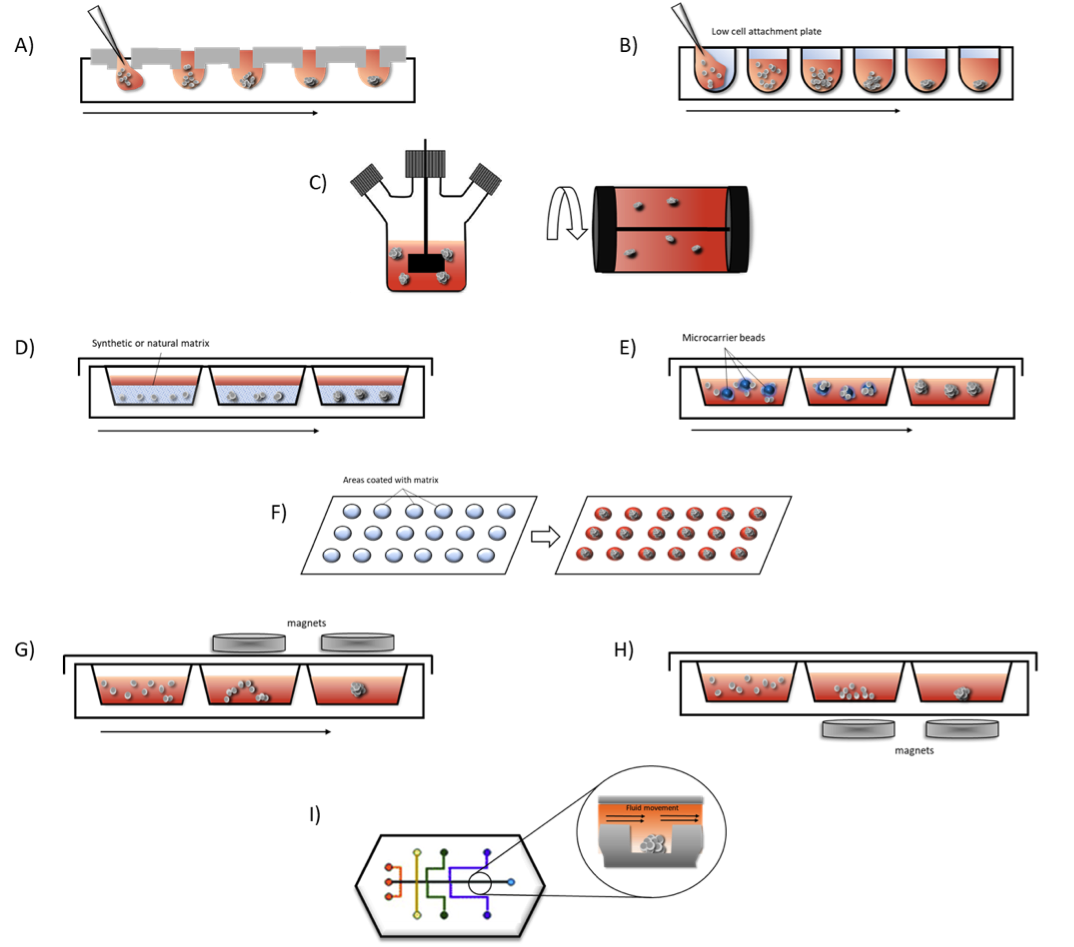
A. Hanging Drop Method – cells are seeded in small droplets of medium and are forced into cellular aggregates due to gravity.
B. Low cell attachment plates – surfaces are modified to promote cellular aggregation into spheroids rather than cell adhesion to cultureware surface.
C. Spinner flasks – stirred or rotating vessels prevent cell adhesion to flasks through continued agitation promoting spheroids formation in suspension.
D. Matrices – cells are seeded into natural or synthetic matrices and scaffolds allowing spheroids to form on these frameworks.
E. Microcarrier beads – cells adhere to and proliferate on the surface of natural or synthetic solid beads coated with matrices that allow cells to adhere and proliferate to form spheroids.
F. Micropatterned plates – plates are etched with matrices promoting spheroid formation in these areas.
G. Magnetic levitation – cells are magnetized using nanoparticles and incubated under magnetic forces, which causes the cells to be suspended within the culture to form spheroids.
H. Magnetic bioprinting – magnetized areas are printed onto the bottom of cultureware using magnetic forces. This method can be used to assemble cells in different 3D patterns, such as cylindrical or honeycomb.
I. Microfluidics – cell spheroids situated in microchannels in a perfusion system allowing for flow of nutrients and oxygen to the cells and waste to be removed simulating vasculature found in organ systems.
Challenges
Despite their contribution to many areas of research, 3D cultures are not without their challenges. Perhaps the biggest challenge, and a very practical one to most researchers, is the unfamiliar and complexed workflows of 3D cell culture and analysis. It is a huge undertaking to even the most skilled lab scientists to achieve reproducibility and spheroid size uniformity in the 3D cultures.
Compared to other techniques, the scaffold-free systems have been found to be much less intimidating and more easily adaptable to the existing cell culture routine by researchers. Advances in low cell attachment culture vessels, such as the Nunclon™ Sphera™ 3D culturewares (Thermo Fisher Scientific, Inc.) coated with polymers to minimize surface variability, improve the consistency and reproducibility of spheroid formation have made it easier for researchers. The Nunclon Sphera microplates, in particular, reduce the occurrence of unwanted satellite colonies enabling single spheroid formation in each well with size control through the initial cell seeding density, lending themselves more easily for use in HTS workflows19 (Figure 3). Size uniformity is particularly important for obtaining highly reproducible results in drug assays because cellular functions within spheroids is strongly linked with size6,20.
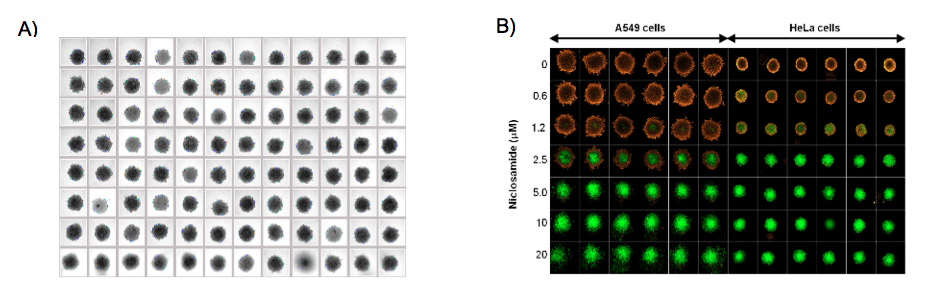
A. Bright field image of A549 human lung 3D tumor spheroids with uniform size and shape in the 96 wells.
B. Niclosamide-induced mitochondrial membrane depolarization and apoptosis in A549 and HeLa 3D tumor models. Tumor spheroids are double stained with MitoTracker Orange and CellEvent Green Caspase 3/7
With respect to scaffold-based techniques, the type of scaffold selected for spheroid formation can have a huge impact on the uniformity and quality of the spheroids formed. To date, more than 100 types of matrices and scaffolds have been developed, most of which are optimized specifically for the growth of the cell type under investigation17, therefore, care must be taken to select the one for your research needs. Poor reproducibility with biomimetic scaffolds has been reported, affecting the consistency of spheroid formation, and the nonhuman origin of some of these scaffolds may limit their utility in human regenerative or transplantation therapy applications21.
Another challenge is releasing the spheroids from the scaffold itself. Natural polymers can be enzymatically digested and synthetic scaffolds often require toxic chemicals to break chemical bonds, which can have a negative effect on spheroid health requiring their removal prior to use in subsequent applications4. Alternative thermal sensitive polymers like Poly(-N-isopropylacrylamide) (PNIPAM) hydrogels have gained popularity because spheroids can be easily extracted by simply altering the culture temperature, without toxic or potent chemicals4.
As described, current 3D culture methodologies are diverse, resulting in spheroids that vary in terms of size, morphology, and complexity. This leads to challenges in obtaining standards with respect to culture and assay protocols, and output data for any given cell type17. Much effort is still needed to ensure reproducibility, high throughput analysis capacity, compatibility of readout techniques, and better automation, to establish standardized and validated 3D cell models8. Particularly in drug screening and preclinical protocols, more comparative data is needed between 2D and 3D cultures to increase confidence in the predictive power of the technology17,20.
Taken together, the desire to use more physiologically relevant 3D spheroid models for in vitro testing, and the need to develop low-cost, standardized, automated, scalable 3D systems are driving innovations for both the scaffold-free and scaffold-based technologies to improve the quality, consistency and predictive capacity of these cultures.
Footnotes
-
1. Fang, Y., & Eglen, R. M. (2017). Three-Dimensional Cell Cultures in Drug Discovery and Development. SLAS Discov. 22(5): 456-472.
-
2. Kunz-Schughart, LA, Freyer, JP, Hofstaedter, F., & Ebner, R. The use of 3-D cultures for high-throughput screening: the multicellular spheroid model. (2004). J Biomol Screen. 9(4): 273–285. doi: 10.1177/1087057104265040
-
3. Landsdowne, LE. (2018, Sept). Benefits of Using 3D Cell Models in Drug Discovery. Technology Networks
-
4. Cui, X., Hartanto, Y., & Zhang H. (2017). Advances in multicellular spheroids formation. J R Soc Interface. 14(127): 20160877. doi: 10.1098/rsif.2016.0877
-
5. Sant, S., & Johnston, P. A. (2017). The production of 3D tumor spheroids for cancer drug discovery. Drug Discov Today Technol. 23: 27-36. doi: 10.1016/j.ddtec.2017.03.002
-
6. Gong, X., Lin, C., Cheng, J., Su, J., Zhao, H., Liu, T., Wen, X., … Zhao, P. (2015). Generation of Multicellular Tumor Spheroids with Microwell-Based Agarose Scaffolds for Drug Testing. PloS one, 10(6): e0130348. doi:10.1371/journal.pone.0130348
-
7. Labusca LS. Scaffold Free 3D Culture of Mesenchymal Stem Cells; Implications for Regenerative Medicine. (2015). J Transplant Stem Cel Biol. 2(1): 8.
-
8. Edmondson, R., Broglie, J. J., Adcock, A. F., & Yang, L. (2014). Three-dimensional cell culture systems and their applications in drug discovery and cell-based biosensors. Assay Drug Dev Technol. 12(4): 207-18. doi: 10.1089/adt.2014.573
-
9. Lee, J. M., Park, D. Y., Yang, L., Kim, E. J., Ahrberg, C. D., Lee, K. B., & Chung, B. G. (2018). Generation of uniform-sized multicellular tumor spheroids using hydrogel microwells for advanced drug screening. Scientific reports. 8(1): 17145. doi:10.1038/s41598-018-35216-7
-
10. Vadivelu, RK, Kamble, H., Shiddiky, M., and Nguyen, NT. (2017). Microfluidic Technology for the Generation of Cell Spheroids and Their Applications. Micromachines. 8(4): 94; doi:10.3390/mi8040094
-
11. Gupta, N., Liu, J. R., Patel, B., Solomon, D. E., Vaidya, B., & Gupta, V. (2016). Microfluidics-based 3D cell culture models: Utility in novel drug discovery and delivery research. Bioeng Transl Med. 1(1): 63-81. doi:10.1002/btm2.10013
-
12. Eglen, R. M., & Klein, J.-L. (2017). Three-Dimensional Cell Culture: A Rapidly Emerging Approach to Cellular Science and Drug Discovery. SLAS Discov. 22(5): 453–455. doi:10.1177/2472555217702448
-
13. Yamada, K.M., Cukierman, E. Modeling tissue morphogenesis and cancer in 3D. (2007). Cell. 130(4): 601–610. doi: 10.1016/j.cell.2007.08.006
-
14. Langhans S. A. (2018). Three-Dimensional in VitroCell Culture Models in Drug Discovery and Drug Repositioning. Frontiers in pharmacology, 9: doi:10.3389/fphar.2018.00006
-
15. Achilli, T. M., Meyer, J., & Morgan, J. R. (2012). Advances in the formation, use and understanding of multi-cellular spheroids. Expert Opin Biol Ther. 12(10): 1347-60.
-
16. Hoarau-Véchot, J., Rafii, A., Touboul, C., Pasquier, J. Halfway between 2D and Animal Models: Are 3D Cultures the Ideal Tool to Study Cancer-Microenvironment Interactions? (2019). J. Mol. Sci.19(1): 181. doi: 10.3390/ijms19010181
-
17. Gitschier, H., Dr Ye Fang, Y., and Eglen, RM. (Spring 2017) 3D Cell Culture - A Rapidly Emerging Technique for Drug Discovery.
-
18. Türker, E., Demirçak, N., Arslan-Yildiz, A. Scaffold-free three-dimensional cell culturing using magnetic levitation. (2018). Biomater Sci. 6:1745-1753. doi: 10.1039/c8bm00122g
-
19. Moldovan N. I. (2018). Progress in scaffold-free bioprinting for cardiovascular medicine. J Cell Mol Med., 22(6): 2964-2969. doi: 10.1111/jcmm.13598
-
20. Mehta, G., Hsiao, A. Y., Ingram, M., Luker, G. D., & Takayama, S. (2012). Opportunities and challenges for use of tumor spheroids as models to test drug delivery and efficacy. J Control Release. 164(2):192-204., 164(2), 192-204. doi: 10.1016/j.jconrel.2012.04.045